Happy Valentine's Day! Valentine's Day is a fun time for many, old and young. It's a time to celebrate the loved ones around you, not necessarily just your partner! We've got some great recipes here to help you celebrate this special day. Now, you won't have to worry about what to cook!
If you're alone this Valentine's Day, that doesn't mean you can't celebrate! Treat yourself to a nice dinner to celebrate loving yourself. It might sound corny, but you owe it to yourself to treat yourself. It's not worth sitting around feeling sorry for yourself.
If you have kids, have them help you in the kitchen! Part of loving means making happy memories. Cooking with your kids can really help you all bond together. Plus, you'll have the chance to teach them some important skills that they can use in their adult lives. Let's get into these recipes!
Valentine’s Day Recipes
Our Valentine's Day recipes are the perfect way to spoil those you love with an extra special meal or treat. Start off the day with breakfast in bed for your sweetie with any of the Valentine's Day breakfast recipes.
No time for breakfast? Try one of these savory Valentines themed dinners. From extravagant lobster dishes to simple and tasty pasta, there is a dish for every taste.
For special desserts or treats for the pint-sized Valentines in your life, bake a special heart shaped dessert to share alone or with the entire class at the school Valentine's Day party. Prepare with love and your Valentine's Day is sure to be a success!
Breakfasts
Valentine's Day Breakfasts
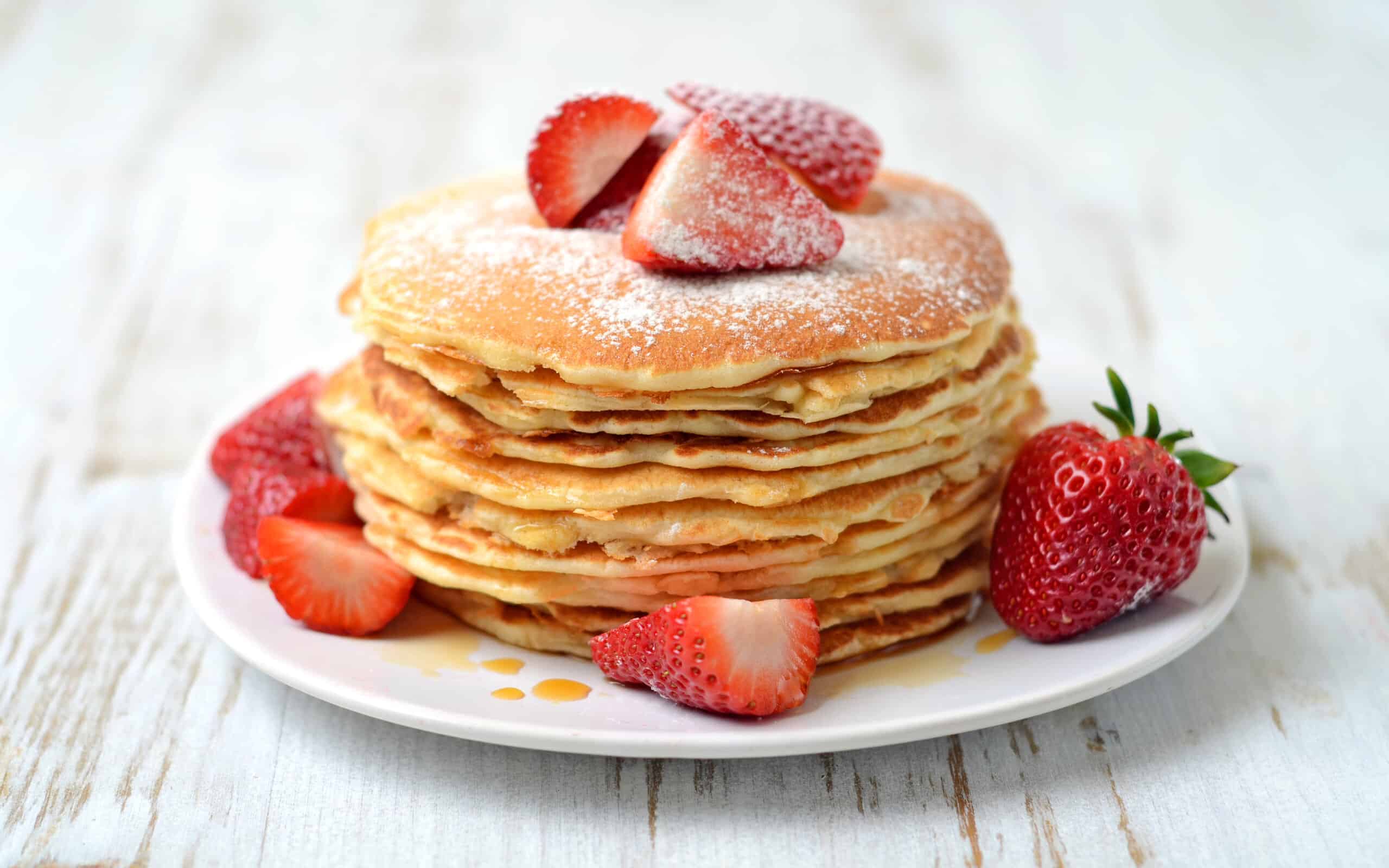
Cheesecake Pancakes & Strawberry-Lemon Syrup
Drinks
Valentine Dinners
Main Dishes
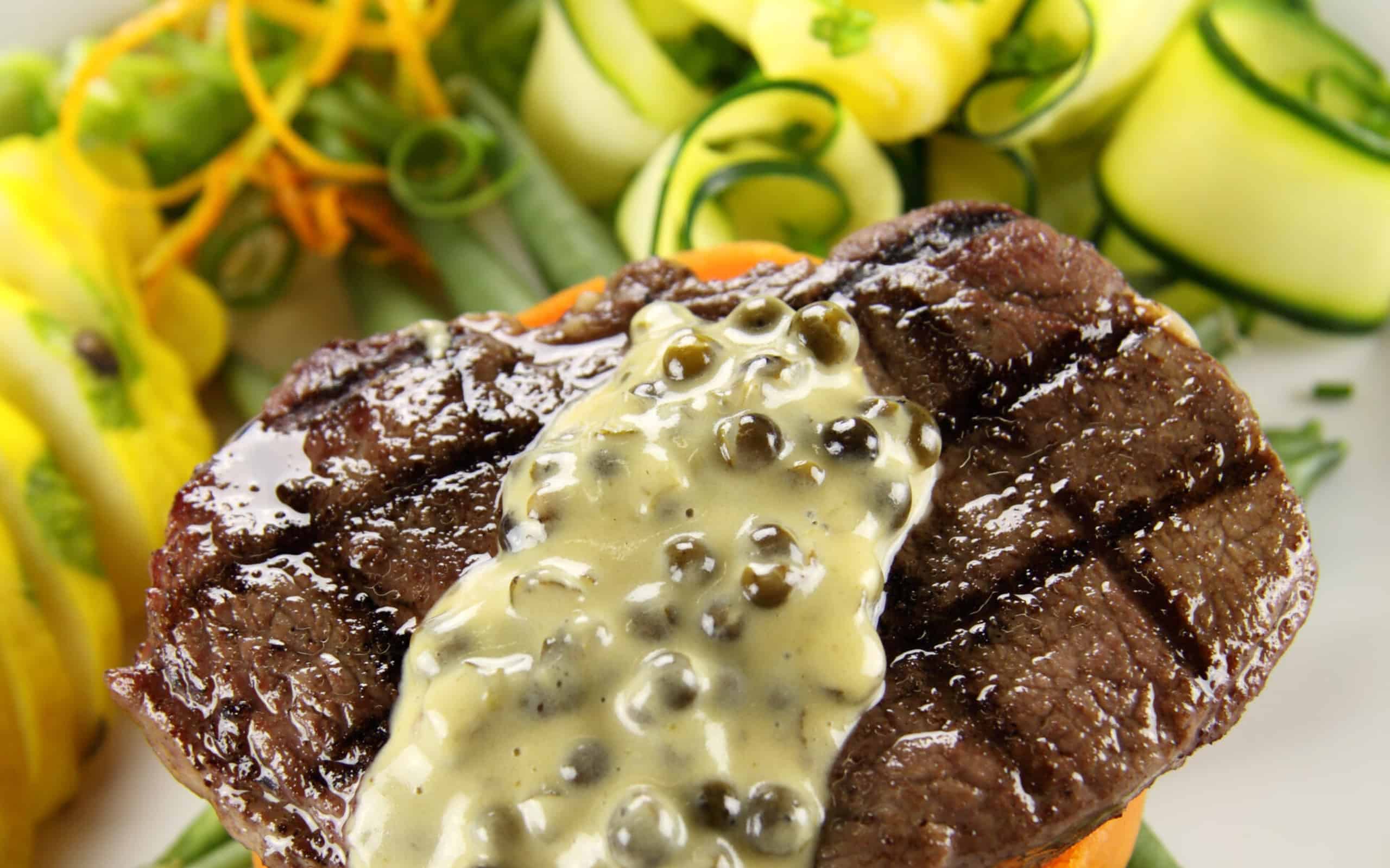
Beef Tenderloin with Peppercorn Sauce
Heart-Shaped Mozzarella and Fontina Pizza
Phyllo-Wrapped Salmon with Roasted Red Peppers
Steak Lover`s Fettuccini Alfredo
Valentine Dessert Recipes
Sweet Treats & Desserts
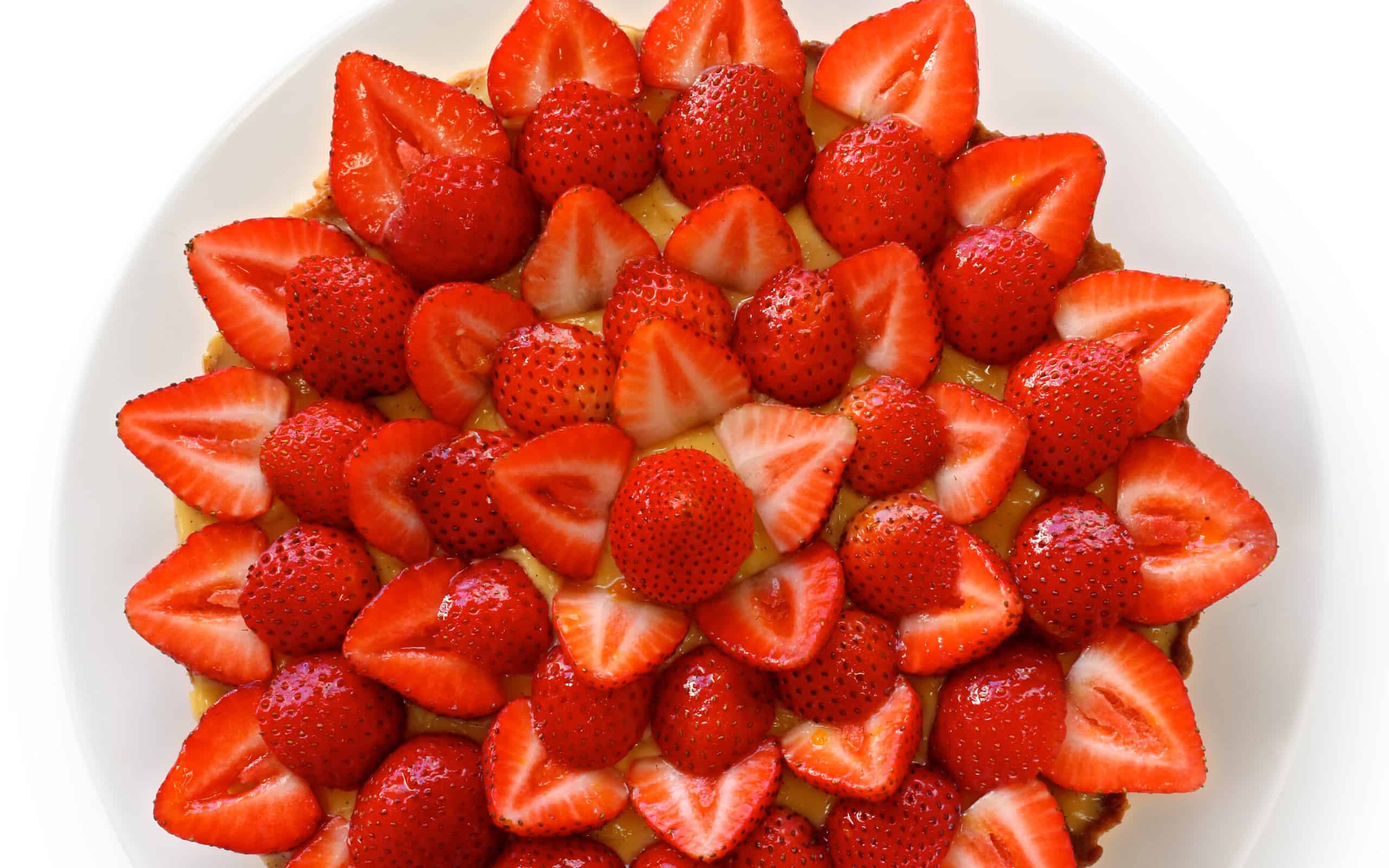
Chocolate Covered Strawberries
Cupid's Cherry Cream Cheese Cookies
Cheesecake Pancakes with Strawberry-Lemon Syrup
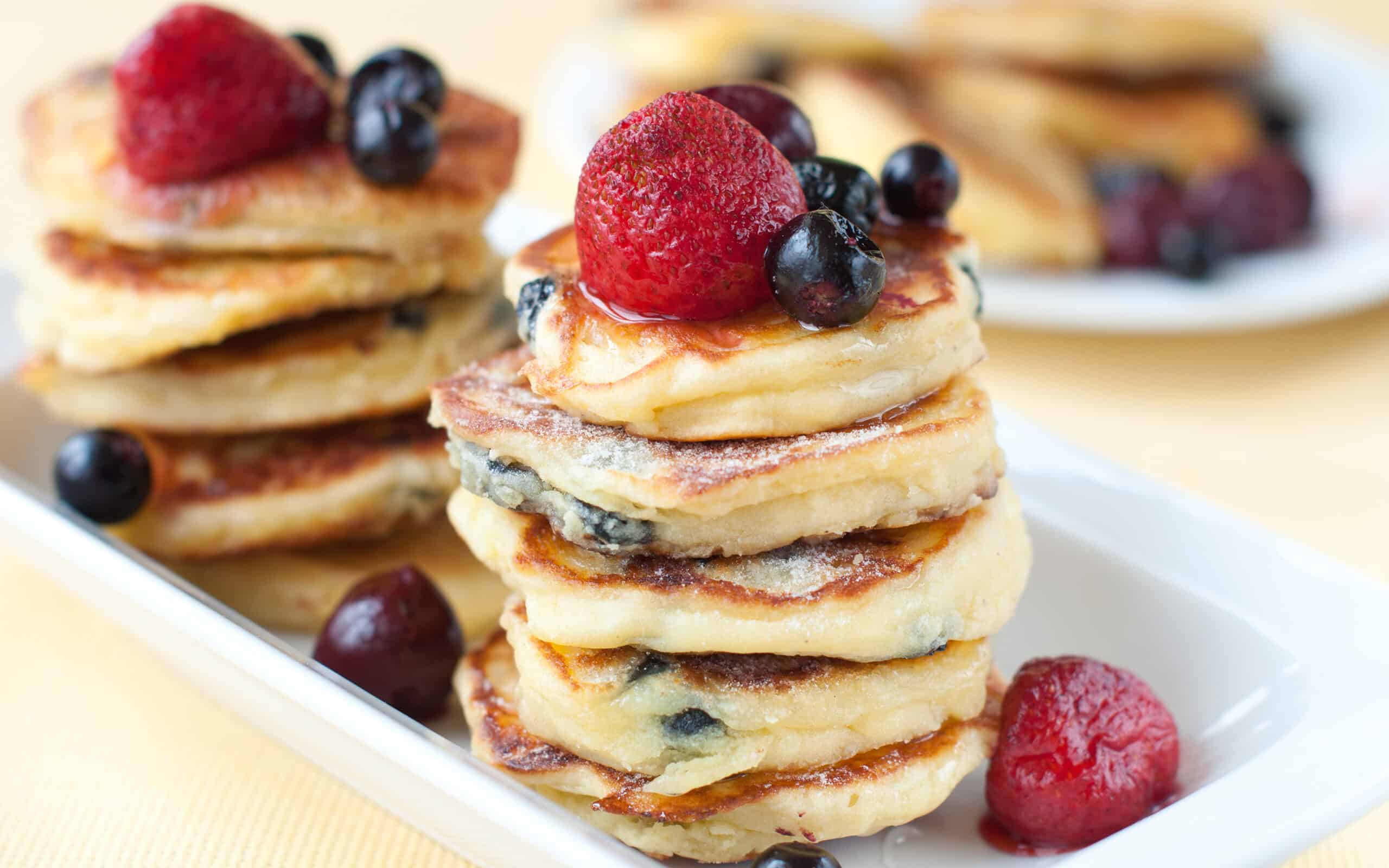
©elenamazur/Shutterstock.com
Ingredients:
* 2 cups Buttermilk pancake mix
* 1 1/4 cup Water
* 1 cup Small curd cottage cheese
* 1 tablespoon Sugar
* 1 teaspoon Vanilla extract
Strawberry-Lemon Syrup
* 1 cup Syrup
* 1 cup sliced strawberries
* 1/2 teaspoon lemon rind
Directions:
Preheat griddle.
Combine the pancake mix, water, cottage cheese, sugar, and vanilla extract in a medium bowl; stir well until blended.
Pour batter by 1/4 cupfuls on hot, well-greased griddle.
Flip pancakes when tops are covered with bubbles.
For Strawberry-Lemon Syrup: Combine the syrup, strawberries, and lemon rind in a small saucepan; heat through, stirring occasionaly.
Cherry French Toast
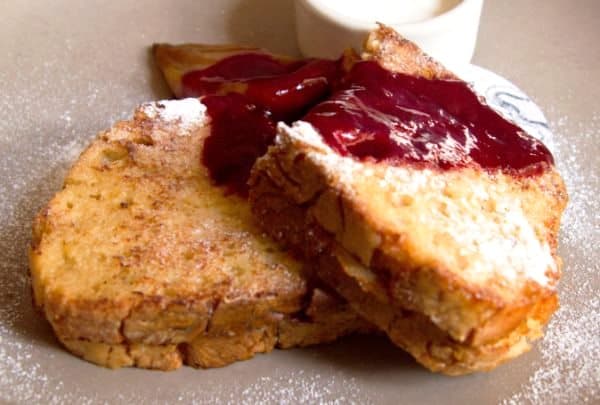
Ingredients:
* 1 1/2 cup milk
* 6 eggs
* 1/8 cup maple syrup
* 3 tablespoons sugar, divided
* 1 tablespoon grated orange peel
* 1/2 teaspoon salt
* 8 slices (4 1/2 x 3 1/2 x 1/2-inch) French bread
* 4 cups pitted Northwest fresh sweet cherries, halved
* 1/2 cup orange juice
* Vanilla yogurt
Directions:
Combine the milk, eggs, maple syrup, 2 tablespoons sugar, orange peel and salt; mix well.
Pour half of mixture into each of two 9-inch square baking dishes. Dip both sides of each slice of bread in milk mixture; arrange in the same baking dish. Cover with plastic wrap and refrigerate overnight.
Marinate the cherries in orange juice and remaining 1 tablespoon sugar overnight.
Carefully transfer slices to a nonstick, buttered baking sheet using a large spatula; allow excess liquid to drip into pan used for soaking.
Bake in a pre- heated 400 degrees F oven 15 to 18 minutes; turn slices over halfway through baking time.
Top each slice with 1/2 cup cherries and a dollop of vanilla yogurt. Serve immediately.
Heart Breakfast Ring
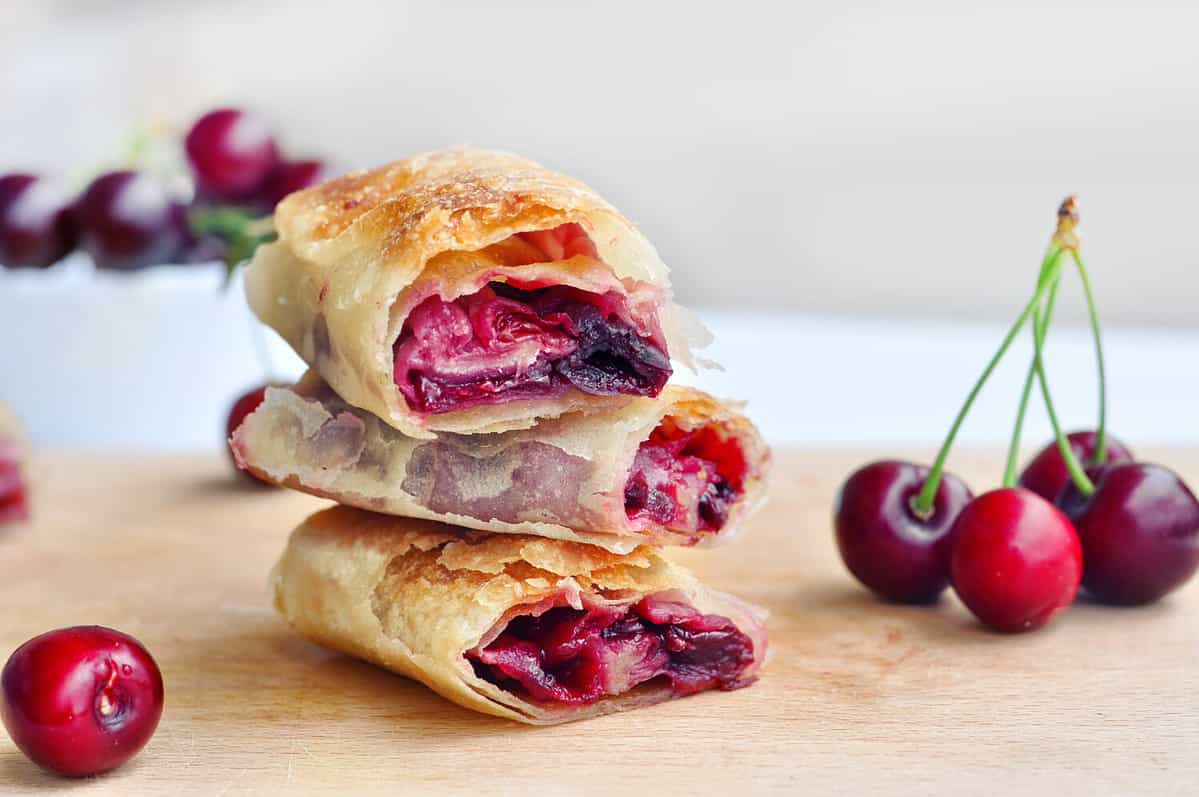
©Minoli/Shutterstock.com
Ingredients:
* 3 cups flour
* 1 package active dry yeast
* 1/4 sugar
* 1 teaspoon salt
* 1/4 cup softened butter or margarine
* 3/4 cup hot tap water
* 1 egg
Filling:
* 1/2 cup flaked coconut
* 1/2 cup finley chopped or sliced almonds
* 3 tablespoons sugar
* 3 tablespoons softened butter or margarine
* 1/2 teaspoon almond extract
* 1/4 cup finely snipped maraschino cherries
Cherry Icing:
* 1/2 cup powdered sugar
* 1 tablespoon maraschino cherry juice
Directions:
Combine 1 cup flour, undissolved yeast, 1/4 cup sugar and salt in large bowl. Stir well. Add 1/4 butter. Add hot tap water.
Beat with electric mixer 2 minutes. Add egg and 1/2 cup flour. Beast until thick and elastic. Gradually stir in just enough flour with wooden spoon to make soft dough that leaves sides of bowl. Turn out onto floured board.
Knead 5-10 minutes. Cover with plastic then a towel. Rest 20 minutes.
Filling: Combine all ingredients except cherries. Mix well.
Punch down dough and roll into a 14×8 rectangle. Spread filling and roll-up tightly beginning with 14″ side.
Place in a ring, seam side down, on greased baking sheet. Seal edges tightly. Snip dough into fourteen 1″ section, cutting from edge of circle to within 1 inch of center. Turn two cut sections on their sides in opposite directions to form a heart. Repeat until you have a circle of 7 hearts.
Put snipped cherries into each heart. Lightly Brush dough with oil. Refrigerate 2 to 24 hours.
When ready to bake, uncover and let stand 10 minutes. Bake at 375 degrees F for 20 – 25 minutes. Use lower rack. If browning too quickly, lower heat to 350 degrees F. Remove from baking sheet immediately.
Combine powdered sugar and cherry juice in a small bowl. Outline hearts with cherry icing.
Strawberry Stuffed French Toast
Ingredients:
* non-stick cooking spray
* 12 slices bread
* 1/2 cup cream cheese, softened
* 1/2 cup strawberry fruit spread
* 1/2 cup sliced strawberries
* 2 large eggs
* 1/2 cup milk
* 1 teaspoon vanilla extract
* 2 tablespoons powdered sugar
* 1/2 cup maple syrup (optional)
Directions:
Spray a large nonstick skillet with cooking spray; set aside.
Lay 6 slices of bread on your work surface. Keeping edges free of food, place a generous teaspoon of cream cheese, a generous teaspoon of fruit spread, and 4 or 5 strawberry slices on each piece. Top with remaining bread.
In a medium mixing bowl, combine eggs, milk, and vanilla. Dip sandwiches, being careful not to let strawberries fall out. Press edges together to seal.
Heat skillet to medium. Cook each French toast sandwich 2 or 3 minutes or until the first side is golden brown.
Use a spatula to turn sandwich over, and cook until second side is golden brown. Sprinkle with powdered sugar.
Serve immediately with maple syrup, if desired.
Valentine's Morning Breakfast
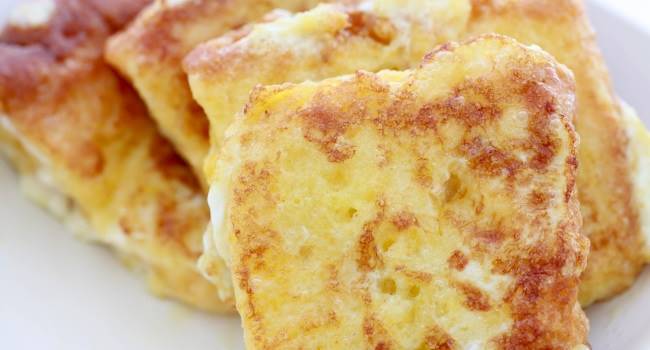
©Benny-K/Shutterstock.com
Ingredients:
* 8 ounces cream cheese, softened
* 1 teaspoon vanilla extract
* 1/2 cup chopped walnuts
* 1 (16 ounce) loaf French bread
* 4 eggs
* 1 cup whipping cream
* 1/2 teaspoon vanilla extract
* 1/2 teaspoon ground nutmeg
* 1 (12 ounce) jar apricot preserves
* 1/2 cup fresh orange juice
Peach Champagne Cocktails
* 1 bottle champagne
* Peach Schnapps
Directions:
Beat together the cream cheese and 1 teaspoon vanilla extract until fluffy; stir in nuts and set aside.
Cut bread into 10 to 12 (1 1/2-inch) slices. Cut a pocket in the top of each.
Fill each with 1 1/2 teaspoons of the cheese mixture. Beat together eggs, whipping cream, the remaining 1/2 teaspoon of vanilla and nutmeg.
Using tongs, dip the filled bread slices in the egg mixture, being careful not to squeeze out the filling.
Cook on a lightly greased griddle until both sides are brown. Keep slices warm in oven.
Heat together the preserves and juice, drizzle over hot French bread.
Valentine Muffins
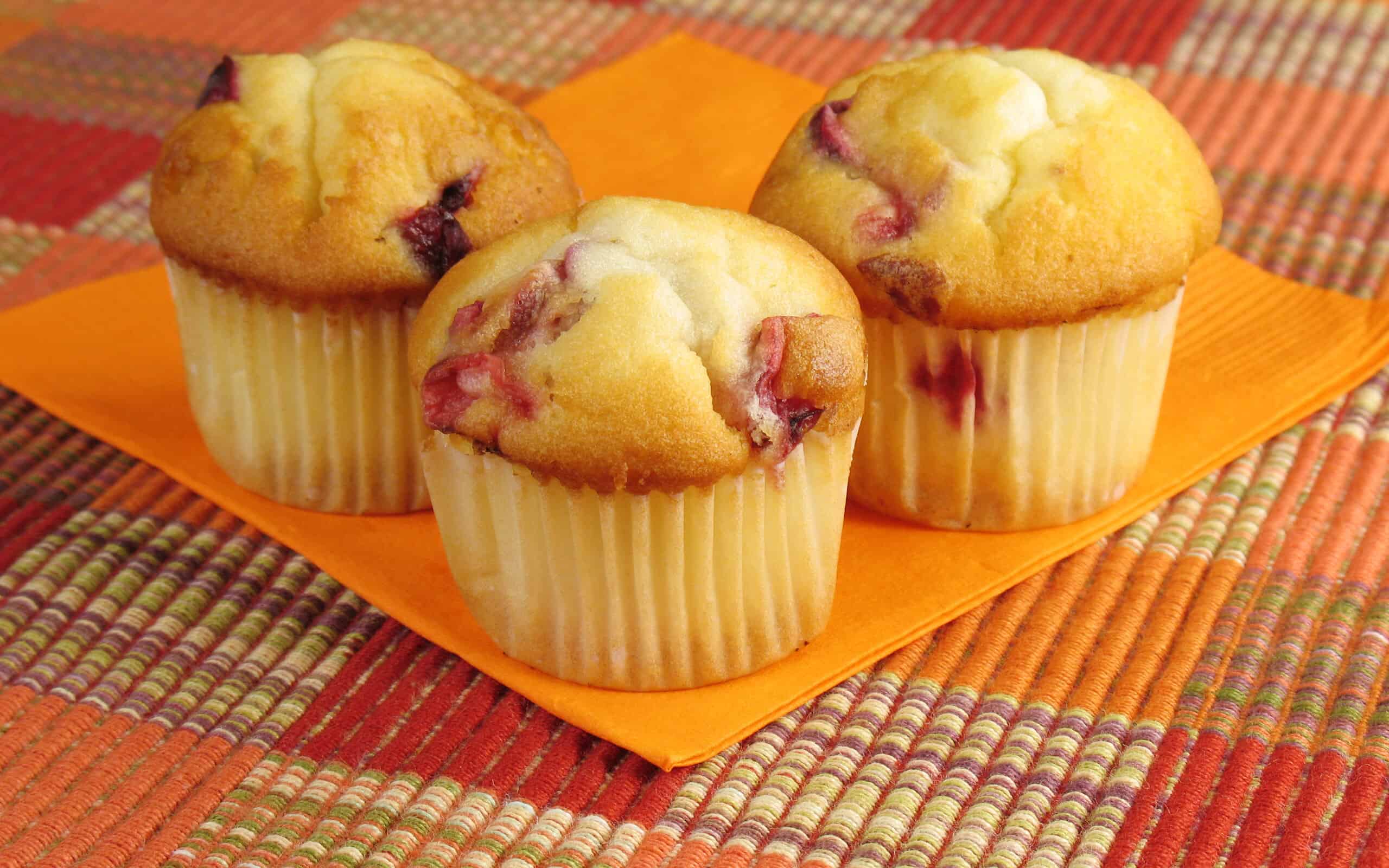
©Andrea Skjold Mink/Shutterstock.com
Ingredients:
* 6 tablespoons butter
* 3/4 cup sugar
* 2 eggs
* 1/2 cup milk
* 14 strawberries, fresh or defrosted frozen
* Food coloring, optional
* 2 cups all-purpose flour
* 1/4 teaspoon salt
* 1 tablespoon baking powder
* Hershey's Kisses, Hugs or strawberry jam
Directions:
Preheat the oven to 350 degrees F. In a large bowl, cream the butter and sugar.
Mix in the eggs, one at a time, and add the milk.
Rinse the strawberries and cut off the greens with a plastic knife (a fun job for 3- to 5-year-olds who are accustomed to cutting play dough).
Mash the berries with a potato masher or puree in a blender. Stir the berries into the butter and milk mixture.
Tip: For muffins with a more pronounced pink color, add a few drops of red liquid food coloring.
In a separate bowl, sift the flour, salt and baking powder. Stir well.
Add the flour mixture to the berry mixture. Use a wooden spoon to stir until all the white disappears.
Line the muffin tin with paper liners. Drop the batter from a tablespoon to fill the cups halfway.
Add an unwrapped Kiss, Hug or 1/2 teaspoon of jam. Then spoon more batter to fill almost to the top.
Bake until the muffins begin to brown and a toothpick inserted near the center (but not in the Kiss) comes out clean, about 20 to 25 minutes.
Remove the muffins from the tin and cool.
Valentine Pancakes
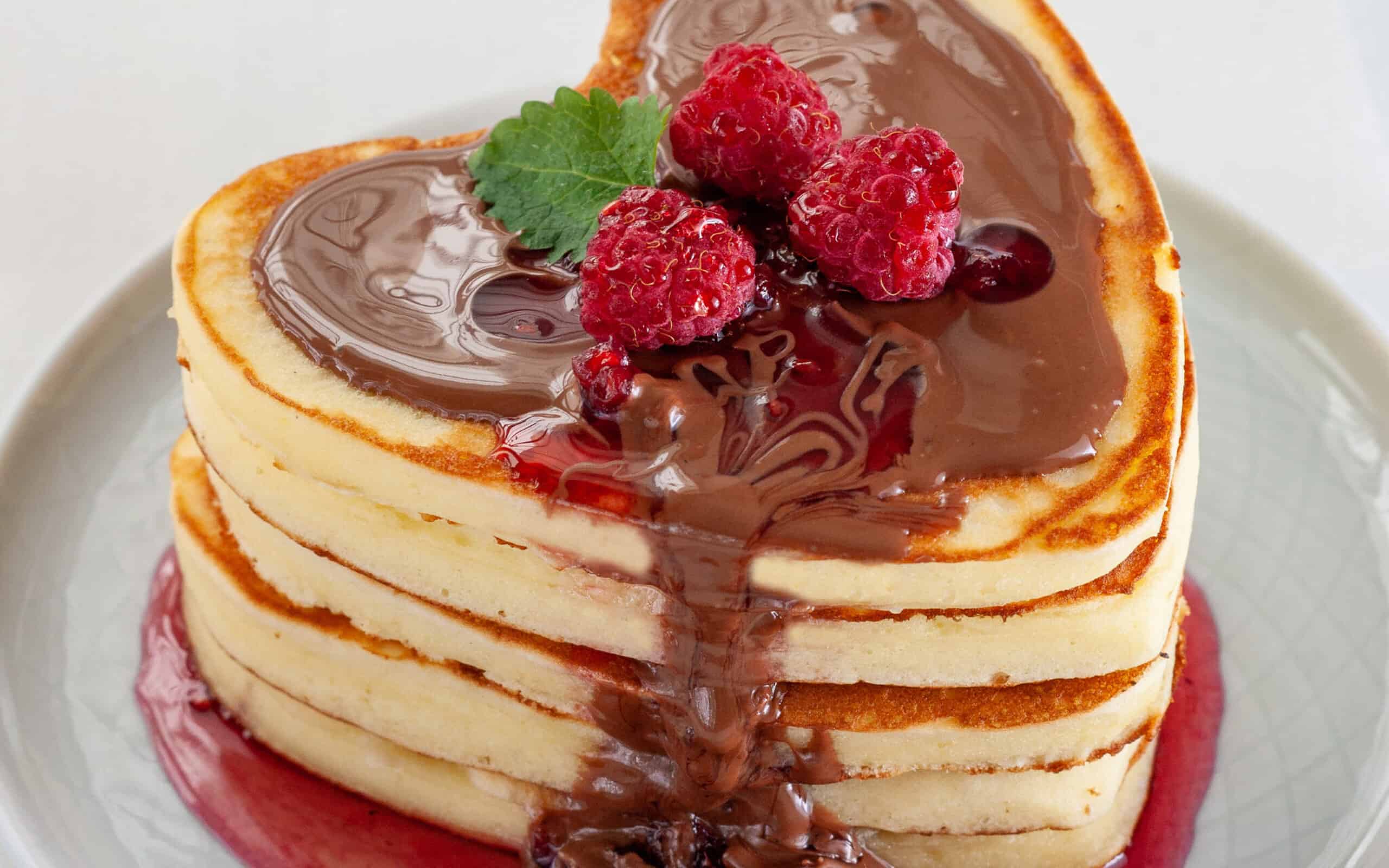
©Mycleverway/Shutterstock.com
Ingredients:
* 2 cups cake flour
* 2 teaspoons baking powder
* 1 teaspoon salt
* 1/2 cup sugar
* 1 egg
* 1 cup milk
* 1/4 cup shortening, melted
* red food coloring (optional)
Directions:
Measure sifted flour into sifter, add baking powder, salt and sugar.
Beat eggs in bowl. Add milk and blend. Sift dry ingredients into mixture gradually.
Add melted shortening. Beat with mixer. Add a few drops of red food coloring, if using, until batter is desired color (pink or red).
Preheat a skillet over medium heat. Use a pan with a nonstick surface or apply nonstick spray.
Pour the batter by spoonfuls into the skillet, in 6 inch circles.
When the edges start to crisp, flip the pancakes. The cooked side should be golden brown.
Cook pancakes on the other side for same amount of time, until golden brown.
Serve with butter and strawberry syrup.
Love Potion
©Foodpics/Shutterstock.com
Ingredients:
* 1/2 cup frozen strawberries, slightly thawed
* 1/2 cup frozen raspberries, slightly thawed
* 1 cup white grape juice (or apple juice)
* Maraschino cherries, for garnish
Directions:
Place the first 3 ingredients in a blender and mix on High until you have a uniform color.
To make the potion thicker, add more fruit; to make it thinner, add more juice.
Garnish with cherries.
Mock Pink Lady
Ingredients:
* 1 1/2 cups milk
* 2 Tablespoons lemon juice
* 1 Tablespoon grenadine
* 1 Tablespoon sugar, granulated
* ice cubes
Directions:
Measure milk, lemon juice, grenadine, sugar and ice cubes into blender.
Blend until ice is finely crushed. Serve immediately.
Passion Fruit Mimosa Recipe
Ingredients:
* 1 cup chilled Champagne
* 1/2 cup chilled passion-fruit juice
Directions:
Divide Champagne between 2 flutes and top off each with passion fruit juice.
Valentine`s Day Beef Tenderloin with Peppercorn Sauce for Two
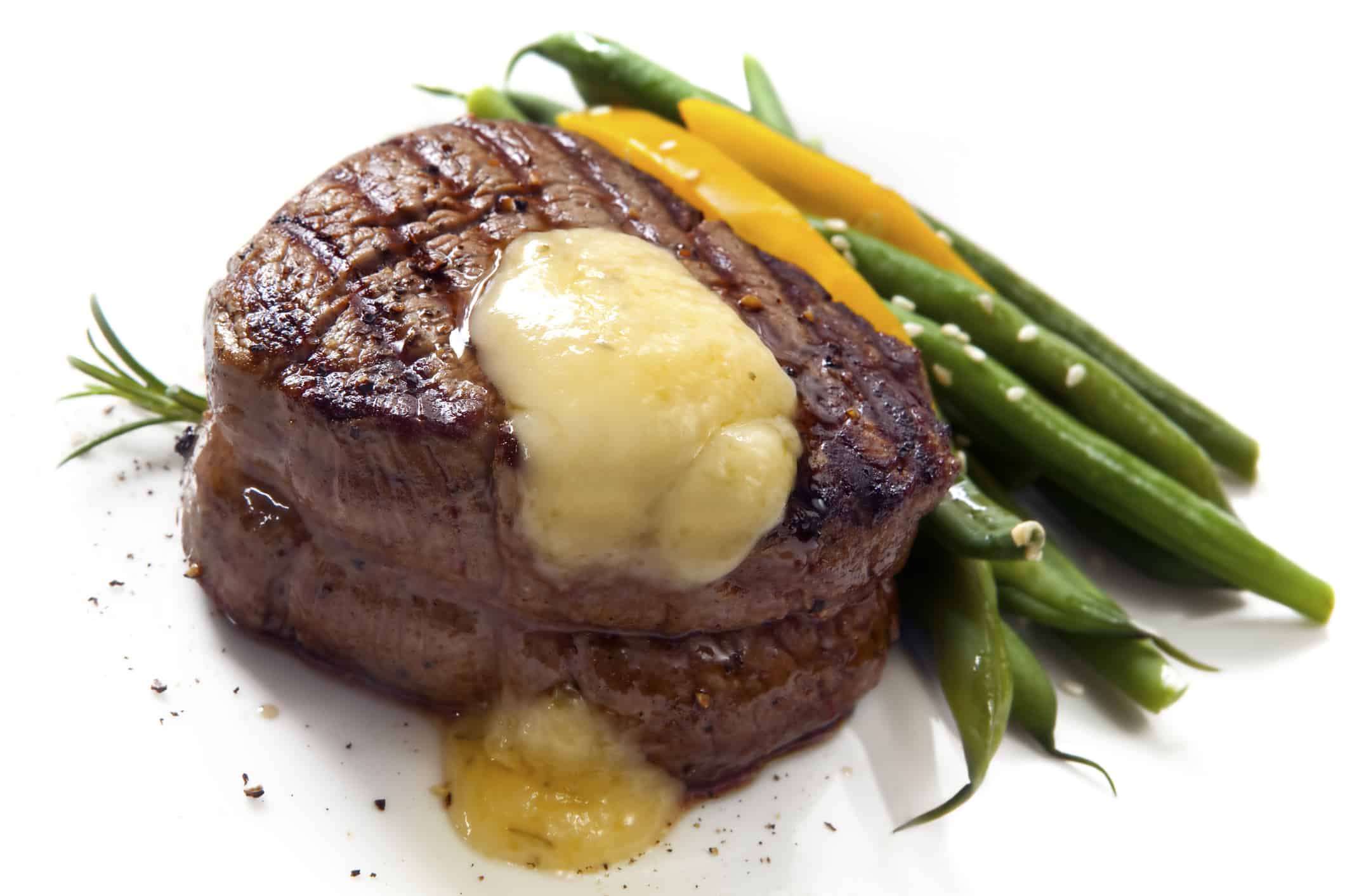
Ingredients:
* 2 (8 oz. ea) beef tenderloins
* salt and pepper
* 1 tablespoon butter
* 3 tablespoons chopped shallots
* 1 Tbl. green peppercorns
* 1 cup beef broth
* 1 tablespoon whole grain Dijon mustard
* 2 tablespoons heavy cream
* 2 tablespoons brandy
* 2 tablespoons chopped chives for garnish
Directions:
Salt and pepper beef on both sides. Heat butter in medium skillet on medium heat.
Add beef; cook 5 min; turn, cook another 5 min. Remove to platter; tent with foil to keep warm.
In same skillet, add shallots and peppercorns. Cook 2 min. or until soft.
Add broth, mustard, cream and brandy. Cook 5 min. or until reduced to 1/2.
Place beef on serving plates. Top with sauce, garnish with chopped chives.
Champagne Shrimp and Pasta
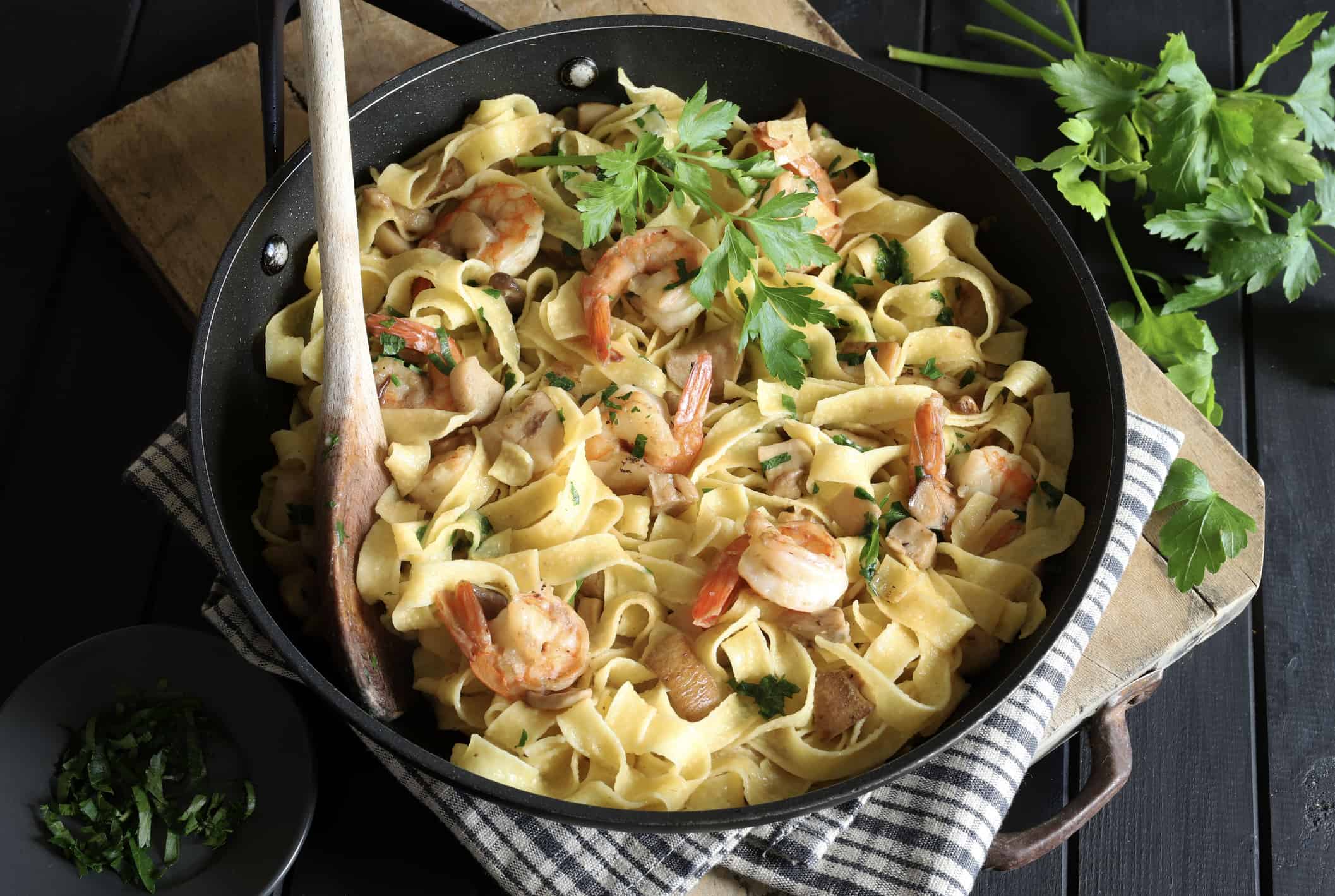
©iStock.com/Grazziela Bursuc
Ingredients:
* 8 ounces angel hair pasta
* 1 tablespoon extra virgin olive oil
* 1 cup sliced fresh mushrooms
* 1 pound medium shrimp, peeled and deveined
* 1 1/2 cup champagne
* 1/4 teaspoon salt
* 2 tablespoons minced shallots
* 2 plum tomatoes, diced
* 1 cup heavy cream
* salt and pepper to taste
* 3 tablespoons chopped fresh parsley
* freshly grated Parmesan cheese
Directions:
Bring a large pot of lightly salted water to a boil. Cook pasta in boiling water for 6 to 8 minutes or until al dente; drain.
Meanwhile, heat oil over medium-high heat in a large frying pan. Cook and stir mushrooms in oil until tender.
Remove mushrooms from pan, and set aside.
Combine shrimp, champagne, and salt in the frying pan, and cook over high heat. When liquid just begins to boil, remove shrimp from pan.
Add shallots and tomatoes to champagne; boil until liquid is reduced to 1/2 cup, about 8 minutes.
Stir in 3/4 cup cream; boil until slightly thick, about 1 to 2 minutes.
Add shrimp and mushrooms to sauce, and heat through. Adjust seasonings to taste.
Toss hot, cooked pasta with remaining 1/4 cup cream and parsley.
To serve, spoon shrimp with sauce over pasta, and top with Parmesan cheese.
Easy Pasta for Two
©
Ingredients:
* 1/2 pound linguini
* 6 Roma Tomatoes – Cut into large dice
* 6 cloves garlic
* 1 tablespoon Extra Virgin Olive oil
* 1/3 cup fresh basil
* 1/2 teaspoon red pepper flakes (or to taste)
* 1/2 teaspoon salt
* Few turns of fresh ground pepper
Directions:
Heat oil in a medium-high skillet, add pepper flakes, garlic, salt and pepper. Cook for 1 minute (don't brown the garlic).
Add the tomatoes and cook until tomatoes start to wilt. Add Basil and cook for 1 more minute.
Add cooked pasta to skillet and toss.
Heart-Shaped Mozzarella and Fontina Pizza
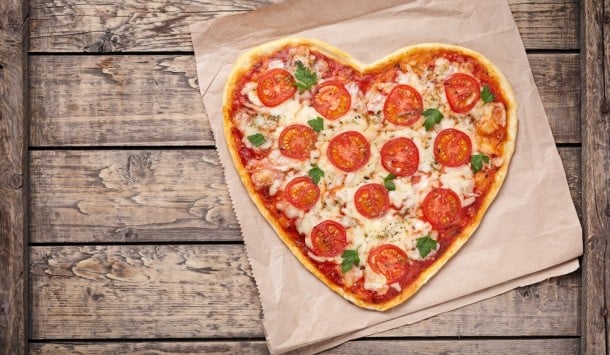
©GreenArt/Shutterstock.com
Ingredients:
* 1 (10 oz.) can refrigerated pizza crust
* 4 teaspoons butter, divided
* 1/2 red pepper, thinly sliced
* 1 leek, cut into 1-inch strips
* 1 boneless, skinless chicken breast half, cut into small cubes
* 1/4 cup pesto
* artichoke hearts, coarsely chopped, to taste
* 4 ounces Fontina cheese, shredded
* 1/2 cup mozzarella cheese, shredded
* 1/2 teaspoon dried oregano
Directions:
Preheat oven to 425 degrees F. Unroll refrigerated pizza dough into rectangle or square shape.
Create a heart shape template out of paper towels or cardboard. Place template on dough and cut around heart shape using scissors.
Place heart-shaped dough on greased cookie sheet and follow instructions on dough can for prebaking pizza crust.
Set crust aside.
Saute red pepper and leeks in half of the butter until almost tender. Remove from skillet.
Add chicken and other half of the butter to skillet and cook until chicken is done and lightly browned.
Spread pesto over prebaked pizza crust. Top with sautéed leeks, red peppers, and chicken. Add artichoke hearts.
Top with cheeses and bake at 425 degrees F. for 7 to 10 minutes. Sprinkle with oregano.
Lobster Scampi
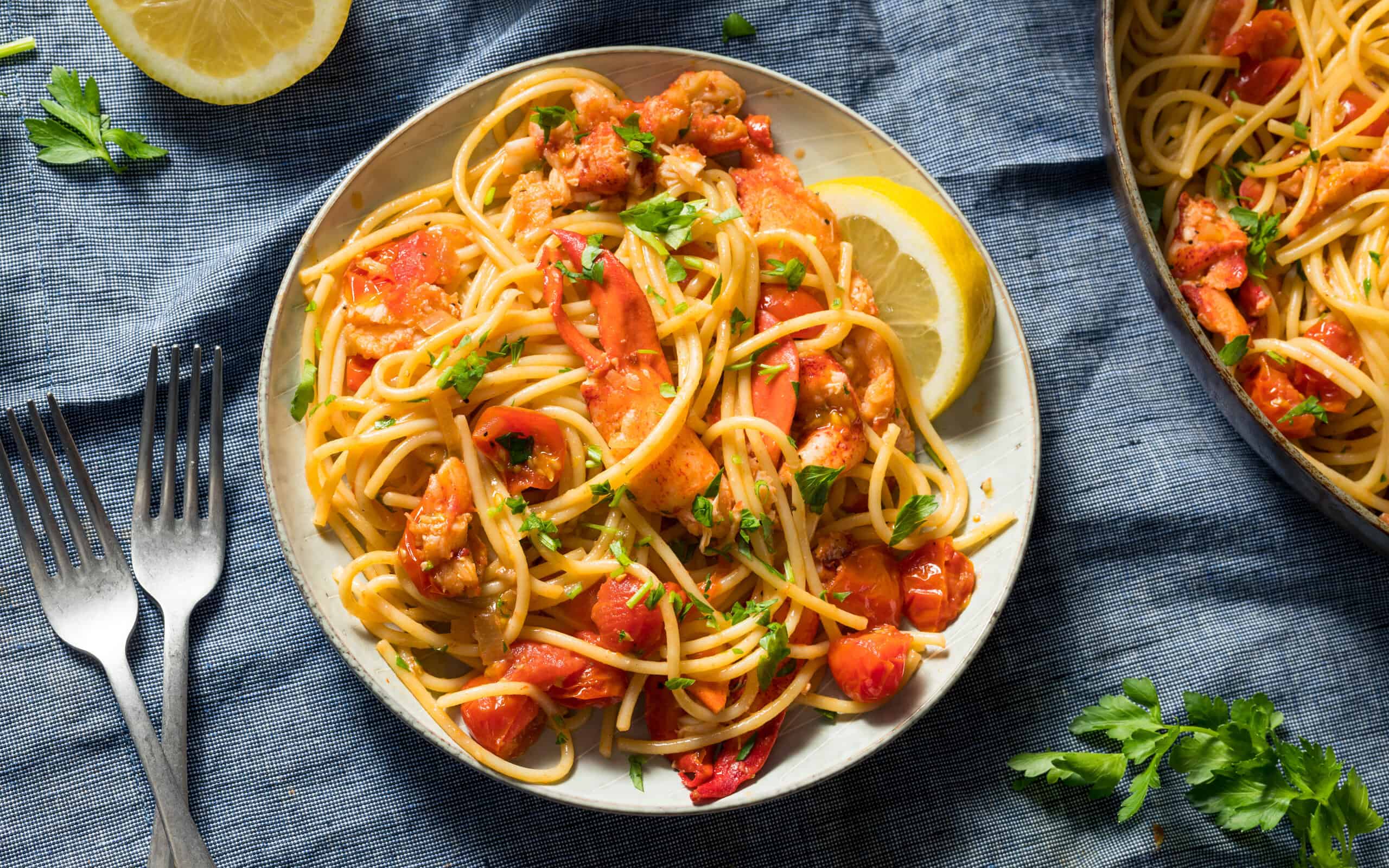
©Brent Hofacker/Shutterstock.com
Ingredients:
* 4 Tablespoons butter
* 1/2 cup garlic olive oil
* 1 Tablespoon hot pepper oil or 1 teaspoon crushed red pepper
* 16 garlic cloves, minced
* 1 Tablespoon Dijon mustard
* 1 teaspoon Hungarian paprika
* 2 Tablespoons Worcestershire sauce
* 1/2 cup dry white wine
* 1 Tablespoon fresh lemon juice
* 1/2 pound cooked lobster meat
Directions:
Melt butter and oils in a large pan over low heat. After it has melted, add the garlic and brown slightly over medium heat.
Whisk in the mustard, paprika, Worcestershire sauce, and wine. Simmer gently for 5 minutes.
Add lemon juice and lobster, and cook until meat is heated through. Serve over yellow rice.
Lobster-Stuffed Tenderloin
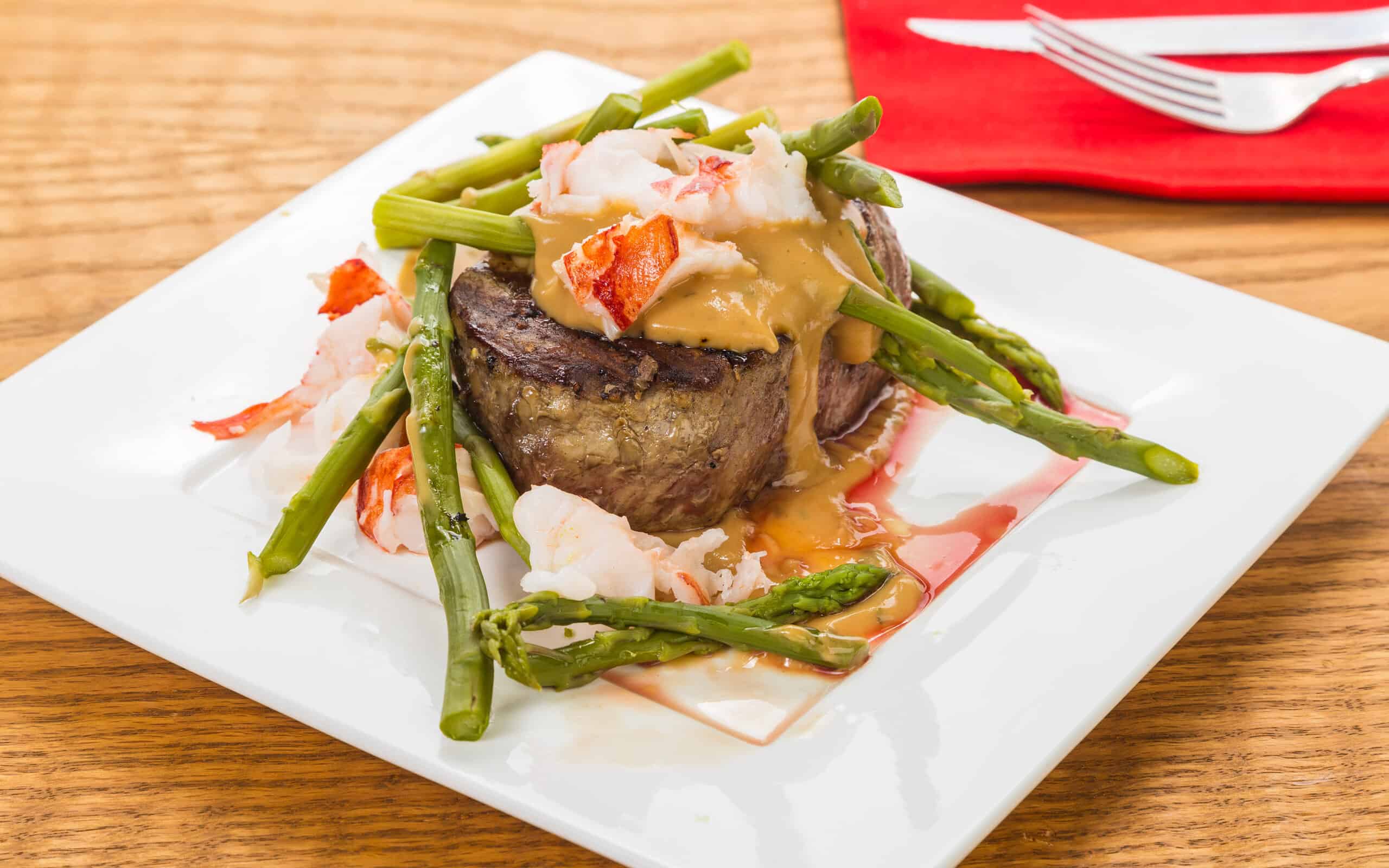
©Warren Price Photography/Shutterstock.com
Ingredients:
* 1 (4 to 5-pound) beef tenderloin
* 1 cup vinaigrette salad dressing
* 1 (2-pound) lobster, cooked or 2 (1-pound) lobsters, cooked
* 6 tablespoons butter or margarine, divided
* 2 tablespoons minced shallots
* 3 tablespoons vermouth
* 1/4 teaspoon dried tarragon, crushed
* 1/2 cup heavy cream
* salt and pepper to taste
Directions:
Place beef tenderloin in a plastic bag set into a shallow dish. Pour salad dressing over tenderloin. Close bag.
Marinate in the refrigerator for 8 to 24 hours, turning bag occasionally.
Remove meat and tomalley (green liver) from lobster. (If desired, reserve shells for use in stock). Cut lobster into bite-size pieces.
In a 10-inch skillet, melt 4 tablespoons of the butter. Add tomalley and cook over low heat for 5 minutes. Add shallots and cook 1 minute more.
Stir remaining butter, vermouth and tarragon into the tomalley mixture.
Cook and stir over medium heat until liquid is reduced to half. Stir in cream.
Continue to cook and stir until thick. Stir in lobster meat. Season with salt and pepper. Set lobster mixture aside.
Preheat oven to 500 degrees F. Drain tenderloin.
To butterfly the meat, make a lengthwise cut down the center of the meat, cutting to within 1 inch of the other side and within 1 inch of ends. Spread meat open slightly and spoon in lobster mixture.
Fold in the thin end portion. Bring sides of tenderloin together and tie with heavy string at 1 1/2-inch intervals to secure.
Place tenderloin on a rack in a shallow roasting pan. Insert a meat thermometer.
Roast, uncovered, for 5 minutes. Reduce oven temperature to 350 degrees F and roast about 30 minutes for rare (140 ).
Let stand 10 to 15 minutes before slicing. Remove string; slice to serve.
Pink Vodka Penne
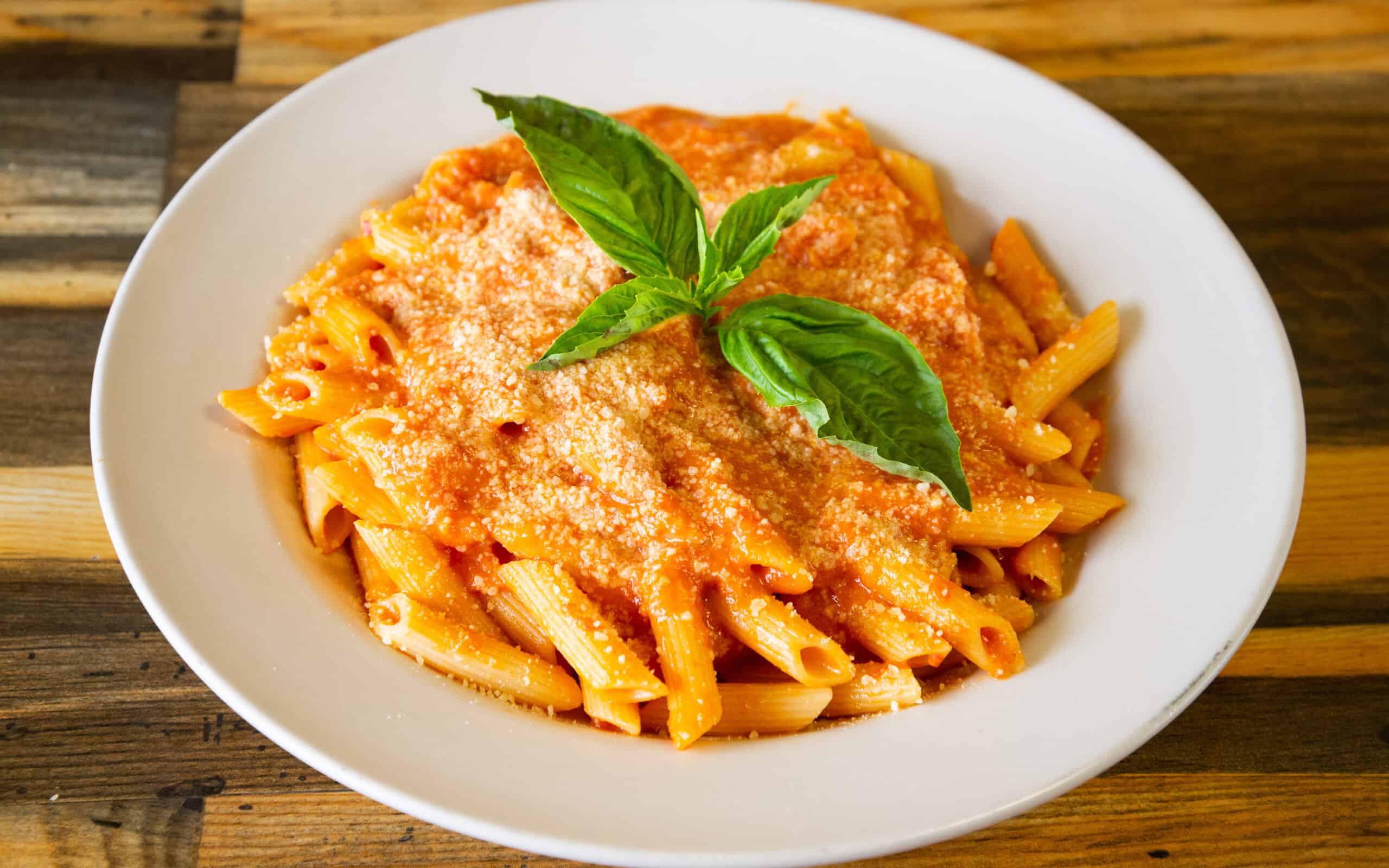
©iStock.com/By Bokey
Ingredients:
* 1 tablespoon extra-virgin olive oil,
* 1 tablespoon butter
* 2 cloves garlic, minced
* 2 shallots, minced
* 1 cup vodka
* 1 cup chicken stock
* 1 can crushed tomatoes (32 ounces)
* Coarse salt and pepper
* 16 ounces pasta, such as penne rigate
* 1/2 cup heavy cream
* 20 leaves fresh basil, shredded or torn
Directions:
Heat a large skillet over moderate heat.
Add oil, butter, garlic, and shallots. Gently saute shallots for 3 to 5 minutes to develop their sweetness.
Add vodka to the pan. Cook until vodka is reduced by half (2-3 minutes).
Add chicken stock and tomatoes. Bring sauce to a bubble and reduce heat to simmer. Season with salt and pepper.
While sauce simmers, cook pasta in salted boiling water until cooked to al dente.
Stir cream into sauce. When sauce returns to a bubble, remove it from heat. Drain pasta.
Toss hot pasta with sauce and basil leaves.
Phyllo-Wrapped Salmon with Roasted Red Pepper
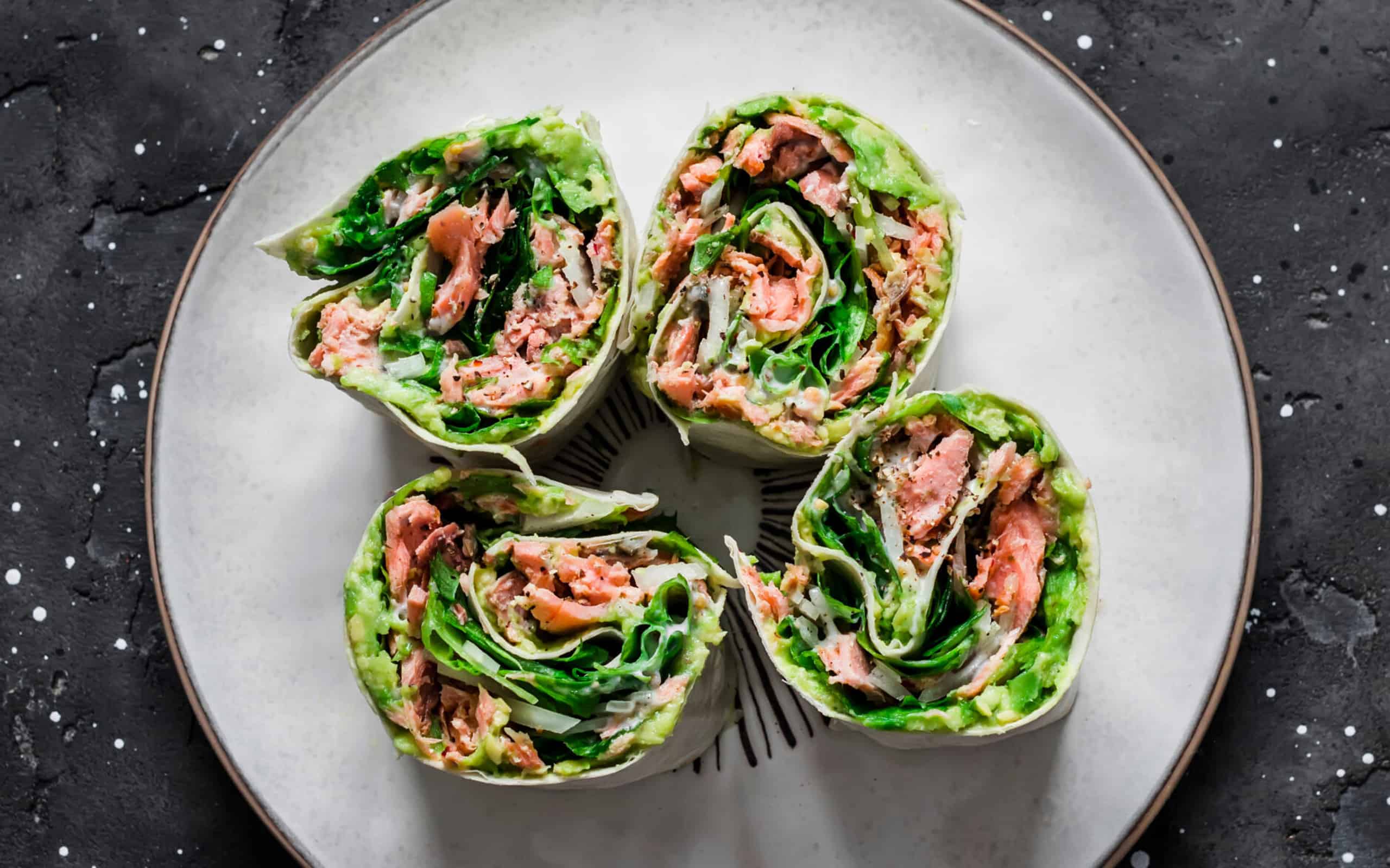
©Kiian Oksana/Shutterstock.com
Ingredients:
* 1 cup roasted red peppers (fresh or jar from Italian market),
* 12 sheets frozen phyllo pastry, thawed,
* 6 tablespoons melted butter or nonstick spray,
* 6 (5 oz.) skinless salmon fillets, 1 inch thick
Directions:
Puree red peppers.
Layer 2 sheets phyllo on work surface, brushing each with butter or spray with nonstick spray; leave remaining sheets of phyllo covered with plastic.
Place 1 fillet crosswise on the pastry, 5 inches from the narrow end. Top with 1 Tbsp. puree.
Fold the 5 inch section of pastry over the salmon and fold in the sides.
Roll the pastry into a rectangular packet to enclose the salmon. Brush with butter on all sides or spray with nonstick spray. Repeat with the remaining pastry and salmon.
Place packets on baking sheet and bake at 400 degrees F for 30-35 minutes until the salmon is cooked through and the pastry is light golden brown. Top with the remaining pepper puree.
Salmon With Cucumber Roses
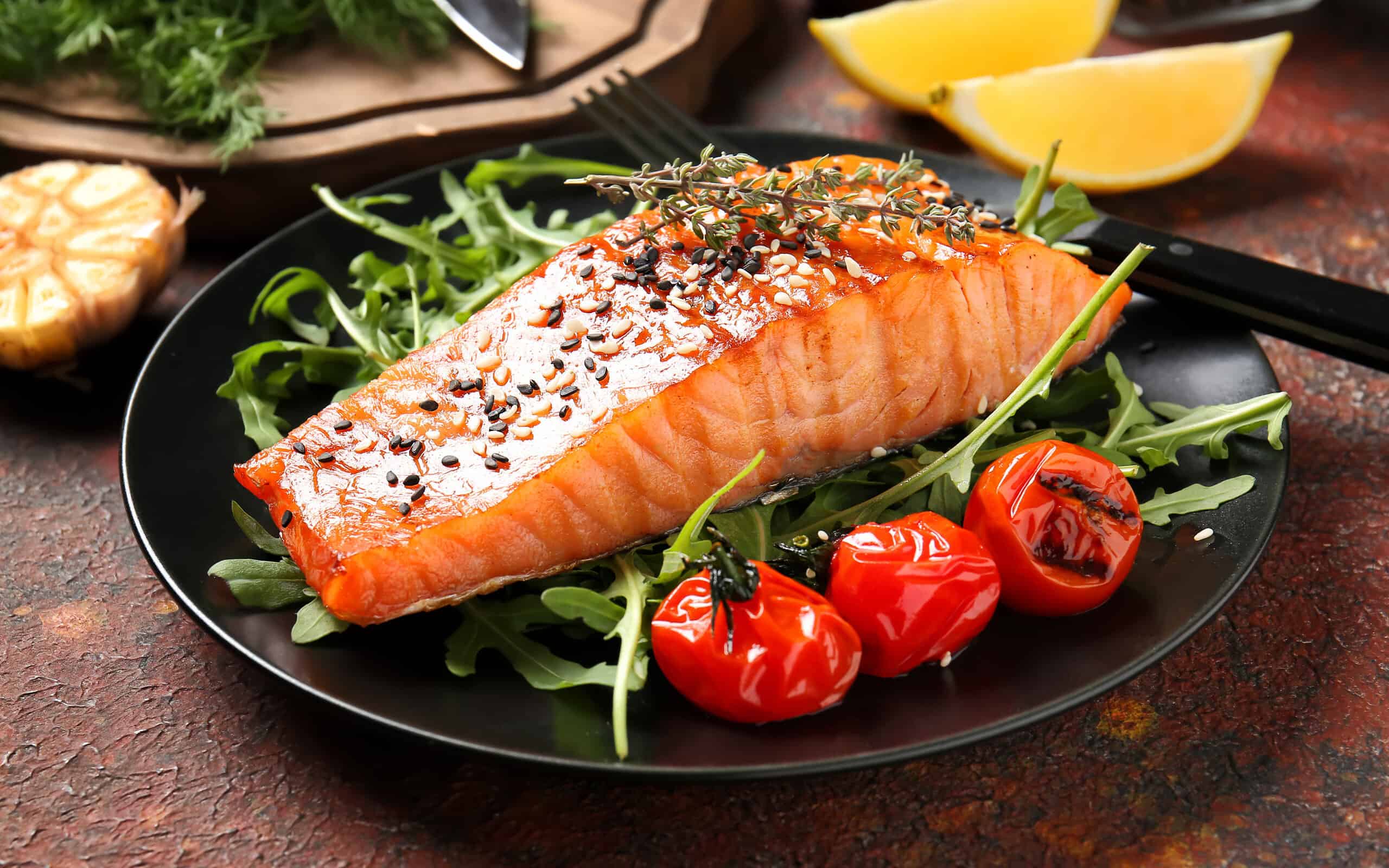
©Pixel-Shot/Shutterstock.com
Ingredients:
* 2 salmon steaks, about 6 ounces each
* salt and pepper
* 2 tablespoons butter
* 1 shallot, chopped
* 1/4 cup vermouth
* 1 English cucumber
* 1/2 cup chopped seeded tomatoes
* 2 tablespoons heavy cream
Directions:
Preheat oven to 425 degrees F. Blot salmon dry and sprinkle with salt and pepper.
Butter oven-proof shallow dish and sprinkle with shallots.
Place salmon in dish, and pour vermouth over fish. Cover with foil and bake for 10 minutes.
Meanwhile, with vegetable peeler, make 6 lengthwise strips of cucumber. Roll each up to form a rose.
Remove salmon from baking dish and cover with foil to keep warm.
Add tomatoes and cream to baking dish and cook over medium heat until slightly thickened.
Steak Lover`s Fettuccini Alfredo
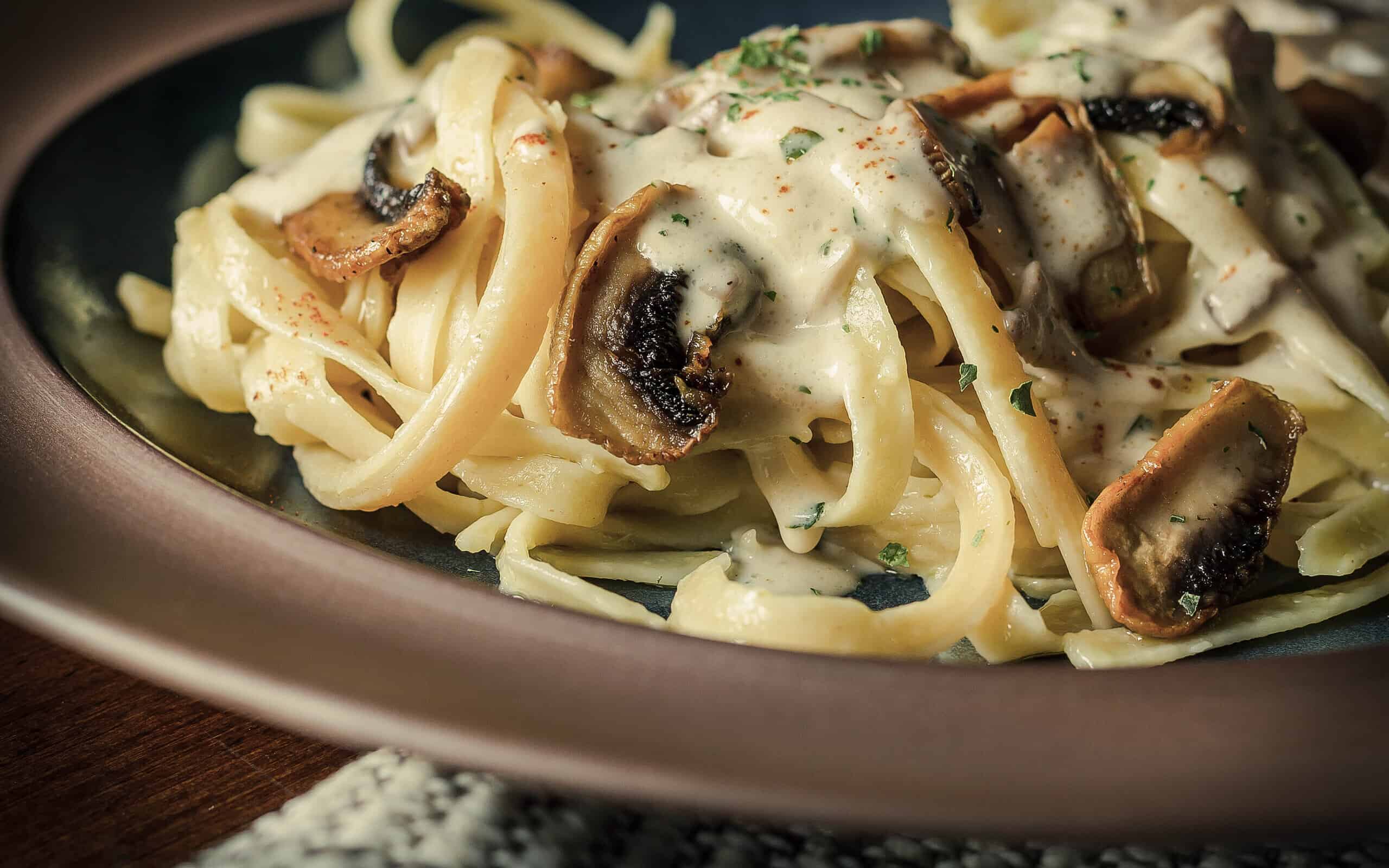
©Ezume Images/Shutterstock.com
Ingredients:
* 1 ounce clarified butter
* 4 ounces beef tenderloin tips
* 1 cup broccoli, red pepper, portabella strips
* 1/4 cup corn, scallion, red and green peppers (small diced)
* 1 tablespoon fresh garlic
* 1 cup heavy cream
* 1/2 cup fresh Parmesan
* 8 ounces cooked pasta
* 1 tablespoon sliced green onion
Directions:
Heat butter. Sear meat and add vegetables.
Add heavy cream and reduce down. Add Parmesan cheese.
When sauce begins to thicken, remove from heat and add to pasta.
Serve hot, topped with scallions and Parmesan cheese.
Lasagna With Prosciutto
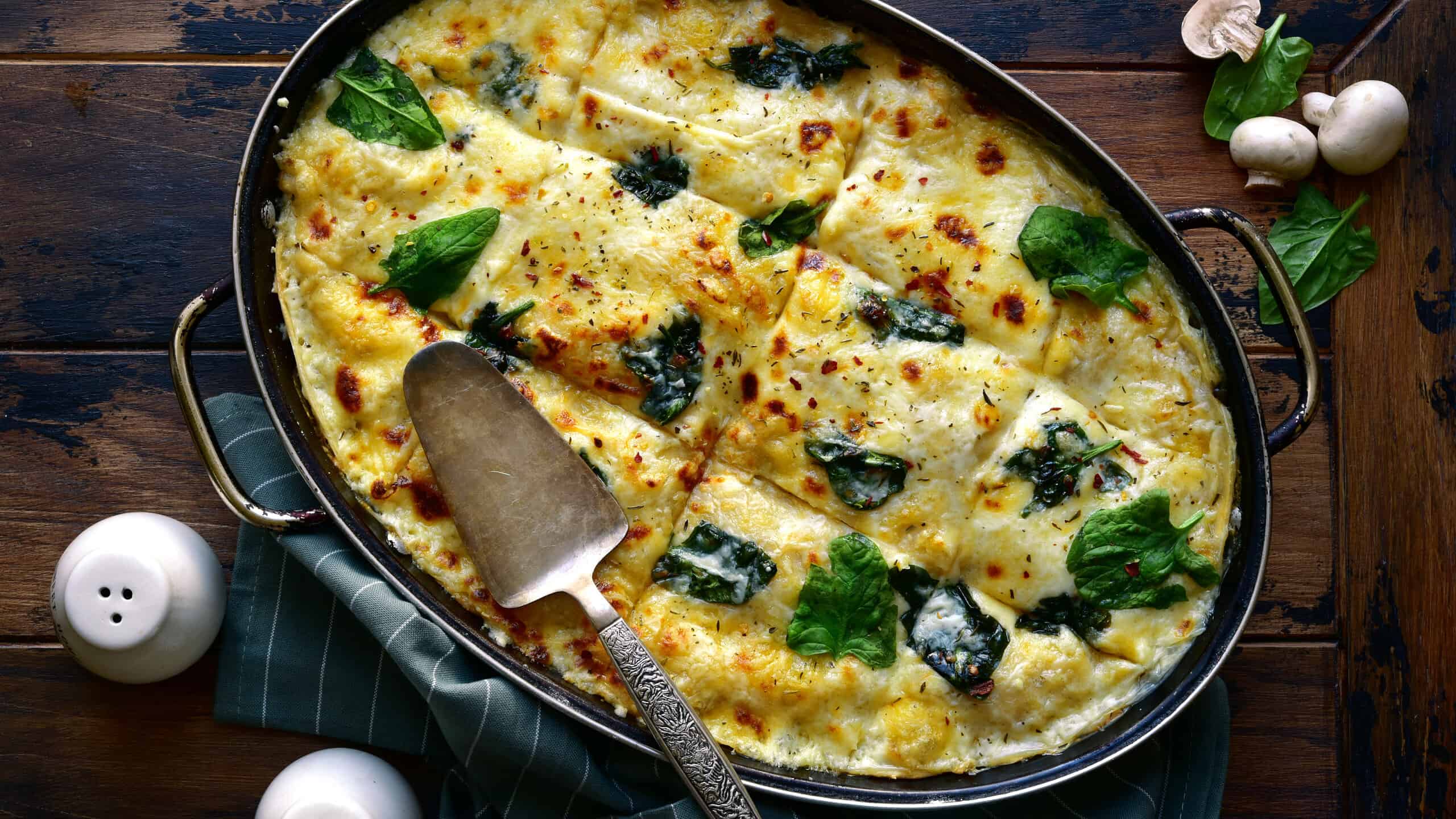
©Liliya Kandrashevich/Shutterstock.com
Ingredients:
8 oz. Italian Prosciutto, chopped
8 oz. Lasagna pasta noodles, cooked
16 oz. can whole peeled tomatoes, chopped
1 cup Ricotta cheese
1 cup grated Parmesan cheese
1 lb. Mozzarella cheese, sliced thin
1 medium onion, chopped
1 lb. lean ground beef
4 Tablespoon fresh basil, chopped
4 cloves garlic, chopped or minced
1/4 teaspoon oregano
ground black pepper
1/2 cup dry white wine
2 Tablespoon olive oil
fresh Basil sprigs for garnish
Directions:
1. Cook lasagna noodles according to package directions or until tender but still firm. Drain, keep warm.
2. In a large saucepan or cast iron skillet, over medium?high high heat, saute the garlic, onion and prosciutto in the olive oil for 4 minutes.
3. Add the lean beef, oregano, a touch of black pepper and saute 10 minutes. Add the wine and cook until the wine is almost evaporated.
4. Add tomatoes and basil, reduce heat and simmer sauce 35 to 40 minutes or until most of the liquid has evaporated.
5. Grease a 9″ x 12″ or larger, baking pan.
6. Arrange a first layer of lasagna noodles, a layer of the beef and prosciutto tomato sauce, Ricotta cheese,
parmesan and mozzarella cheese.
7. Add a second alternating layer of noodles,sauce and other ingredients. Repeat until pan is full ending up with a top layer of sauce.
8. Sprinkle with parmesan cheese and bake in a pre?heated oven at 400 degrees for 30 minutes. Garnish with fresh basil.
Angel Strawberry Bavarian
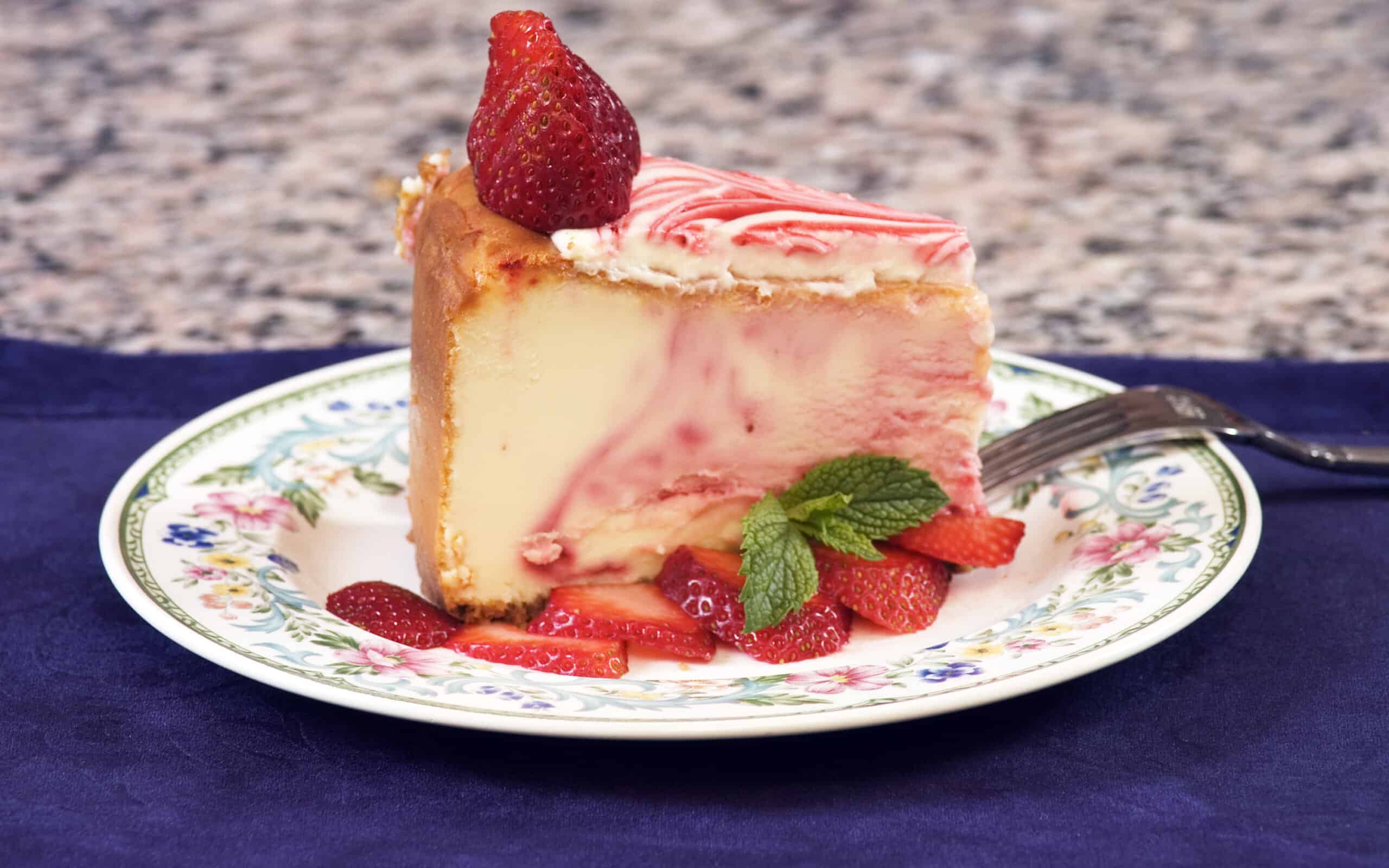
©Jeffrey B. Banke/Shutterstock.com
Ingredients:
* 13 ozs angel food cake, cut into cubes
* 2 1/2 cups Cool Whip® , thawed
* 10 ozs frozen strawberries, reserve syrup
* 2 1/2 tablespoons powdered sugar
* 3 ozs strawberry gelatin powder
* 1 teaspoon pure vanilla extract
* 1 cup boiling water
* 4 whole fresh strawberries, sliced
Directions:
In a small bowl, combine gelatin powder and boiling water. Stir until gelatin has completely dissolved.
Add enough water to strawberry syrup to equal one cup. Stir into gelatin mixture.
Refrigerate until gelatin is slightly thickened.
Beat gelatin until foamy. Fold in one cup whipped topping and frozen strawberries.
Alternate layers of cake cubes and strawberry mixture in 10″ tube pan. Press lightly.
Cover and refrigerate overnight. nmold cake onto serving platter.
Beat remaining whipped topping, powdered sugar, and vanilla extract until stiff peaks form. Frost sides and top of cake.
Refrigerate until ready to serve.
Garnish with fresh strawberries.
Chocolate Cherry Pie
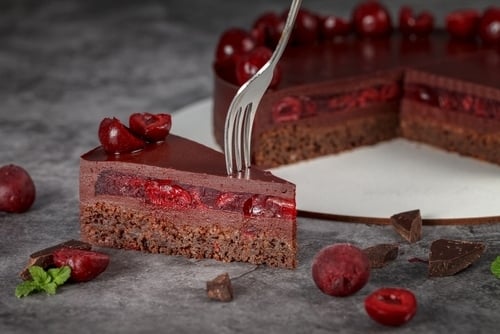
©alex_gor/Shutterstock.com
Ingredients:
* 1 package (8 oz.) cream cheese , softened AND 1 package (3 oz.) cream cheese , softened
* 3/4 cup sugar
* 1 teaspoon vanilla extract
*1/4 cup HERSHEY'S Cocoa
* 2 eggs
* 1/2 cup cold whipping cream
* 1 packaged crumb crust
* 1/2 cup cherry pie filling
Directions:
Heat oven to 350°F.
Beat cream cheese, sugar and vanilla in large bowl until well blended. Add cocoa; beat until well blended, scraping sides of bowl and beaters frequently. Add eggs; beat well. Stir in whipping cream. Pour into crust.
Bake 35 to 40 minutes. (Center will be soft but will set upon cooling). Cool to room temperature. Cover; refrigerate several hours or overnight. Serve topped with cherry pie filling. Cover; refrigerate leftover pie. Makes 8 servings
Cherry Filled Heart Cookies
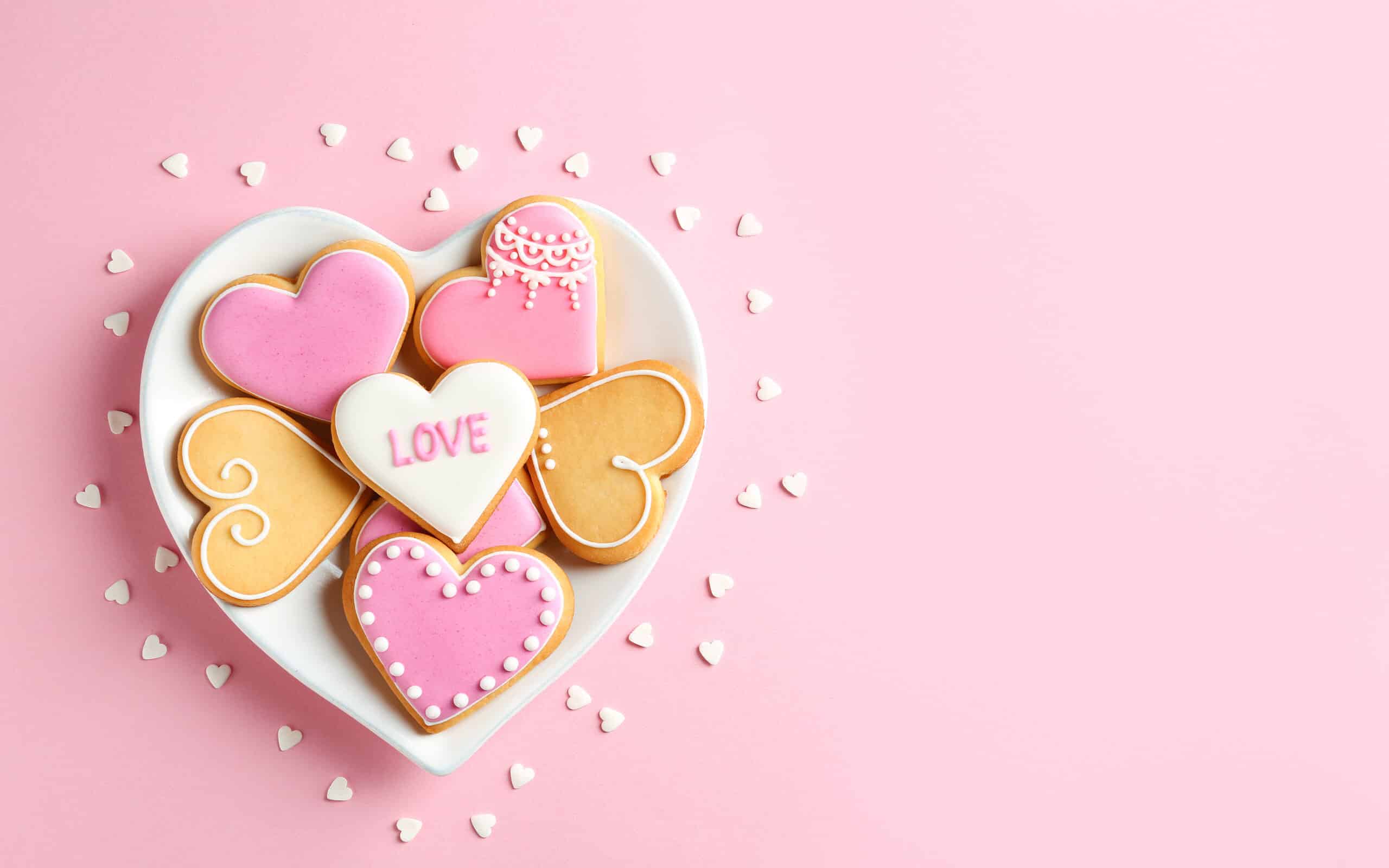
Ingredients:
Cookies:
* 1/2 cup butter or margarine, softened
* 1/2 cup shortening
* 1 cup granulated sugar
* 1 egg
* 1/2 cup milk
* 1 teaspoon vanilla extract
* 3 1/2 cups all-purpose flour
* 2 teaspoons baking powder
* 1 teaspoon baking soda
* 1/2 teaspoon salt
Cherry Filling:
* 1/2 cup granulated sugar
* 4 1/2 teaspoons cornstarch
* 1/2 cup orange juice
* 1/4 cup red maraschino cherry juice
* 12 red maraschino cherries, chopped
* 1 tablespoon butter or margarine
* Additional sugar
Directions:
In a mixing bowl, cream the butter and shortening; gradually add sugar.
Add egg, milk and vanilla extract.
Combine dry ingredients; gradually add to creamed mixture. Mix well.
Cover and refrigerate for at least 2 hours. Meanwhile, make Cherry Filling (directions below).
Roll out dough on a lightly floured surface to 1/8-inch thickness; cut with a 2 1/2-inch heart-shaped cookie cutter dipped in flour.
Place half of the cookies on greased baking sheets; spoon 1/2 teaspoon filling in the center of each.
Use a 1 1/2-inch heart-shaped cutter to cut small hearts out of the other half of the cookies.(Bake small heart cutouts separately.)
Place the remaining hearts over filled cookies; press edges together gently. Fill centers with additional filling if needed. Sprinkle with sugar.
Bake at 375 degrees F for 8 to 10 minutes or until lightly browned. Cool on wire racks.
Yields about 4 1/2 dozen filled cookies.
Cherry Filling Directions:
Combine sugar and cornstarch in a small saucepan.
Add juices, cherries and butter.
Bring to a boil; boil and stir for 1 minutes. Chill.
Cherry Cream Cheese Cookies
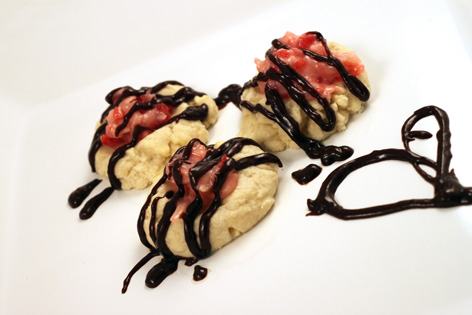
Ingredients:
Cookies:
* 1 cup powdered sugar
* 1 cup butter, softened
* 1 egg
* 2 teaspoons vanilla
* 2 cups flour
Filling:
* 1 cup powdered sugar
* 2 teaspoons flour
* 3 ounces cream cheese
* 1 teaspoon vanilla
* 1/2 cup coconut
* 1/2 cup chopped maraschino cherries — drained
Glaze:
* 1/4 cup chocolate chips
* 1 teaspoon butter
* 1/4 cup powdered sugar
* 3 teaspoons water
Directions:
Mix powdered sugar and butter.
Add egg and vanilla. Stir in flour. Mix until well blended.
Cover and refrigerate for 1 to 2 hours.
Shape dough into balls. Place 2″ apart on cookie sheet.
With thumb, make imprint in each cookie.
Bake for 12 to 15 minutes in a preheated 350 degree F oven. Remove from cookie sheet and cool.
To make filling:
In a large bowl, mix all filling ingredients together until well mixed.
Fill each cookie center with 1/2 teaspoon of filling.
To make glaze:
In saucepan, melt chocolate chips and butter.
Mix in rest of ingredients until smooth and drizzle over cookies.
Melt Away Heart Cookies
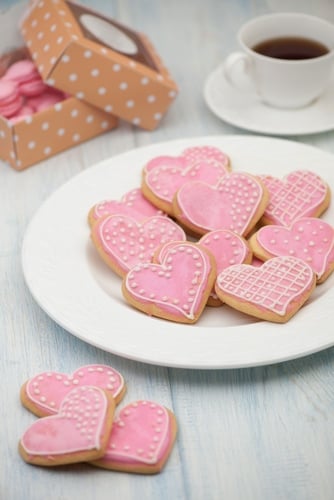
©Alba_alioth/Shutterstock.com
Ingredients:
* 1/3 cup powdered sugar
* 3/4 cup cornstarch
* 1 cup all-purpose flour
* 1/8 teaspoon salt
* 1 cup butter
Cream Cheese Icing
* 3 ounces cream cheese, softened
* 1 tablespoon butter, softened
* 1 tablespoon milk
* 1/2 teaspoon vanilla
* 1/2 pound powdered sugar
* pink food coloring
Cookie Directions:
Sift together dry ingredients. Cut in the butter until fine.
Gently work into a soft dough. Roll dough out onto a lightly floured surface to approximately 1/4-inch thick.
Cut using a 2- or 3-inch heart-shaped cookie cutter. Place hearts onto an ungreased baking sheet.
Bake at 375 degrees F for 7-9 minutes.
Let cookies rest on baking sheet until cooled slightly before removing to cooling rack.
Frost centers of cookies with cream cheese icing.
Cream Cheese Icing Directions:
Mix together cream cheese, butter, milk and vanilla.
Gradually mix in powdered sugar. Add more milk if needed to make a creamy spreading consistency.
Stir in pink food coloring until a pale pink icing is created.
Frost centers of melt-away cookies.
Pink Lemon Fluff Cake
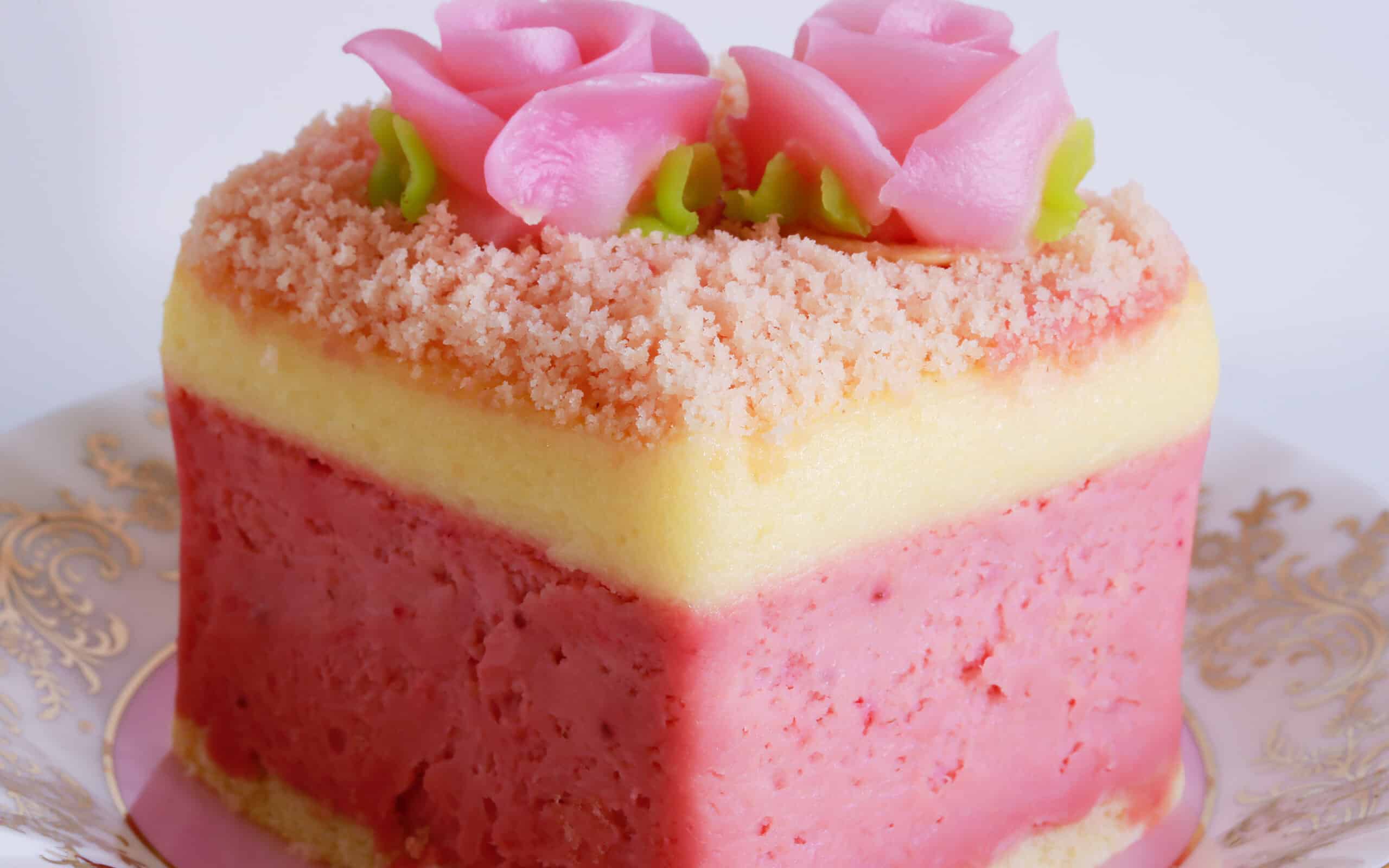
©UNIKYLUCKK/Shutterstock.com
Ingredients:
* 1 cup cake flour
* 3/4 cup sugar
* 12 egg whites, room temperature
* 1 1/2 teaspoon cream of tarter
* 1/4 teaspoon salt
* 1 1/4 teaspoon lemon extract
* 3/4 cup more sugar
* 4 drops red food color (or enough to make pale pink )
Directions:
Sift flour and sugar well.
Combine egg whites with cream of tarter, salt and lemon extract. Beat until foamy.
Beat in food color. Gradually add sugar, 2 Tablespoons at a time, beating until stiff but not dry peaks.
Fold in sugar and flour mixture carefully.
Pour into an ungreased 10 inch tube pan.
Bake in a 325 degree F oven 35 to 45 minutes or until done.
Invert to cool completely before removing.
Pink Glaze
Ingredients:
*2 cups confectioner’s sugar
*3 to 4 tablespoons fresh lemon juice
*Pink food coloring
Directions:
Mix together sugar and lemon juice in a bowl.
Add more sugar or lemon juice as necessary to make glaze thick yet pourable.
Stir in food coloring to get the desired shade of pink.
Queen of Hearts Strawberry Tart
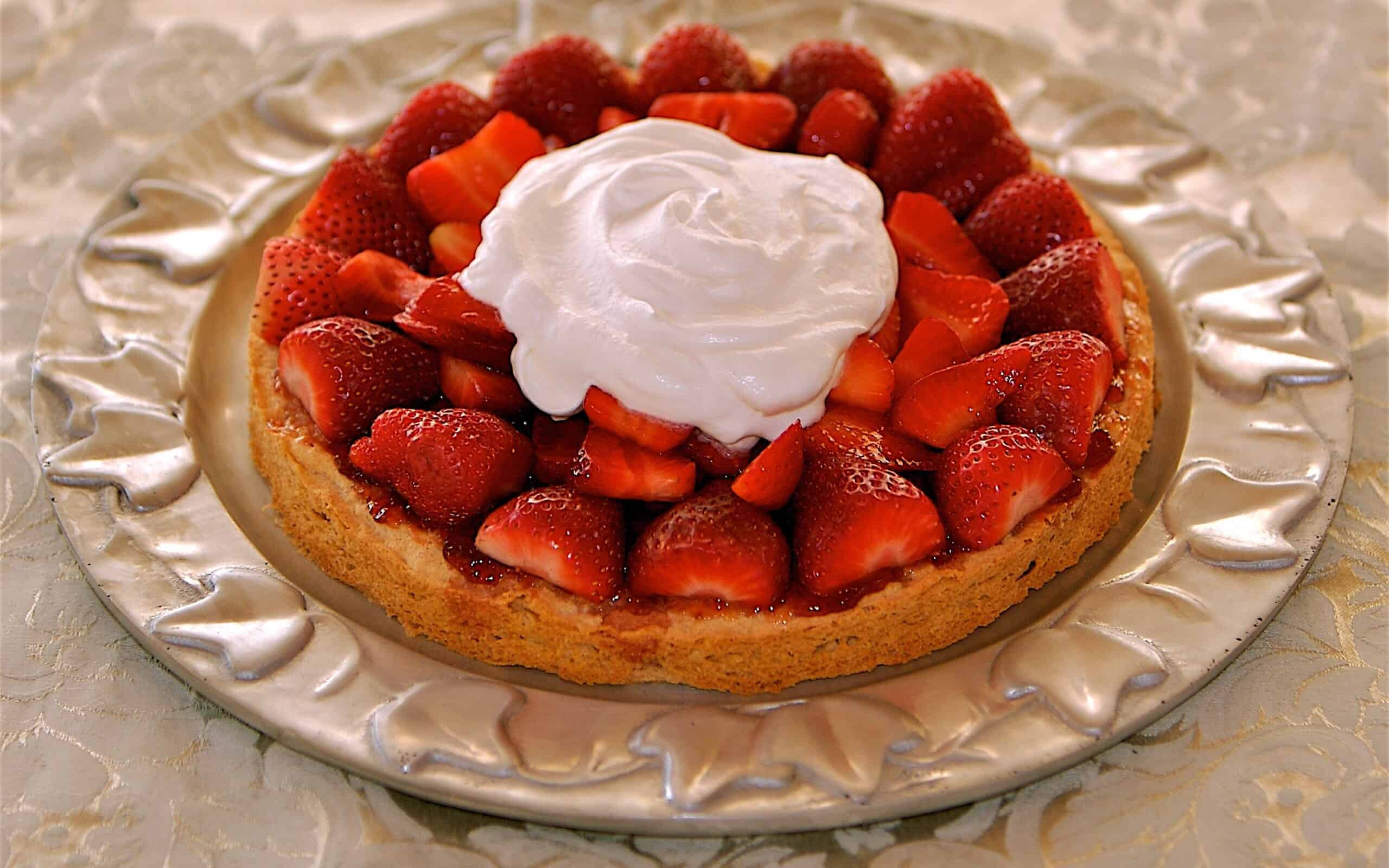
©Tanja Kuic/Shutterstock.com
Ingredients:
Crust:
* 1/2 cup butter, melted
* 1 teaspoon sugar
* 1 cup flour
Filling:
* 4 cups whole strawberries, hulled
* 1 1/2 cup warm water
* 1 1/2 cup sugar
* 4 tablespoons cornstarch
* 1 package (3-ounce size) strawberry gelatin mix
* 2 drops red food coloring
* Whipped cream
Directions:
Preheat oven to 400 degrees F. Mix all crust ingredients and pat into a 9-inch pie plate.
Prick the bottom with a fork and bake for about 15 minutes, or until lightly browned . Cool.
Arrange whole strawberries in the crust.
In a saucepan, cook water, sugar, and corn starch until the mixture becomes clear.
Add gelatin and stir until dissolved. Add food coloring. Pour over the strawberries that are in the crust.
Chill well. Top with whipped cream before serving.
Fresh Strawberries and Cream Filled Brownie Hearts
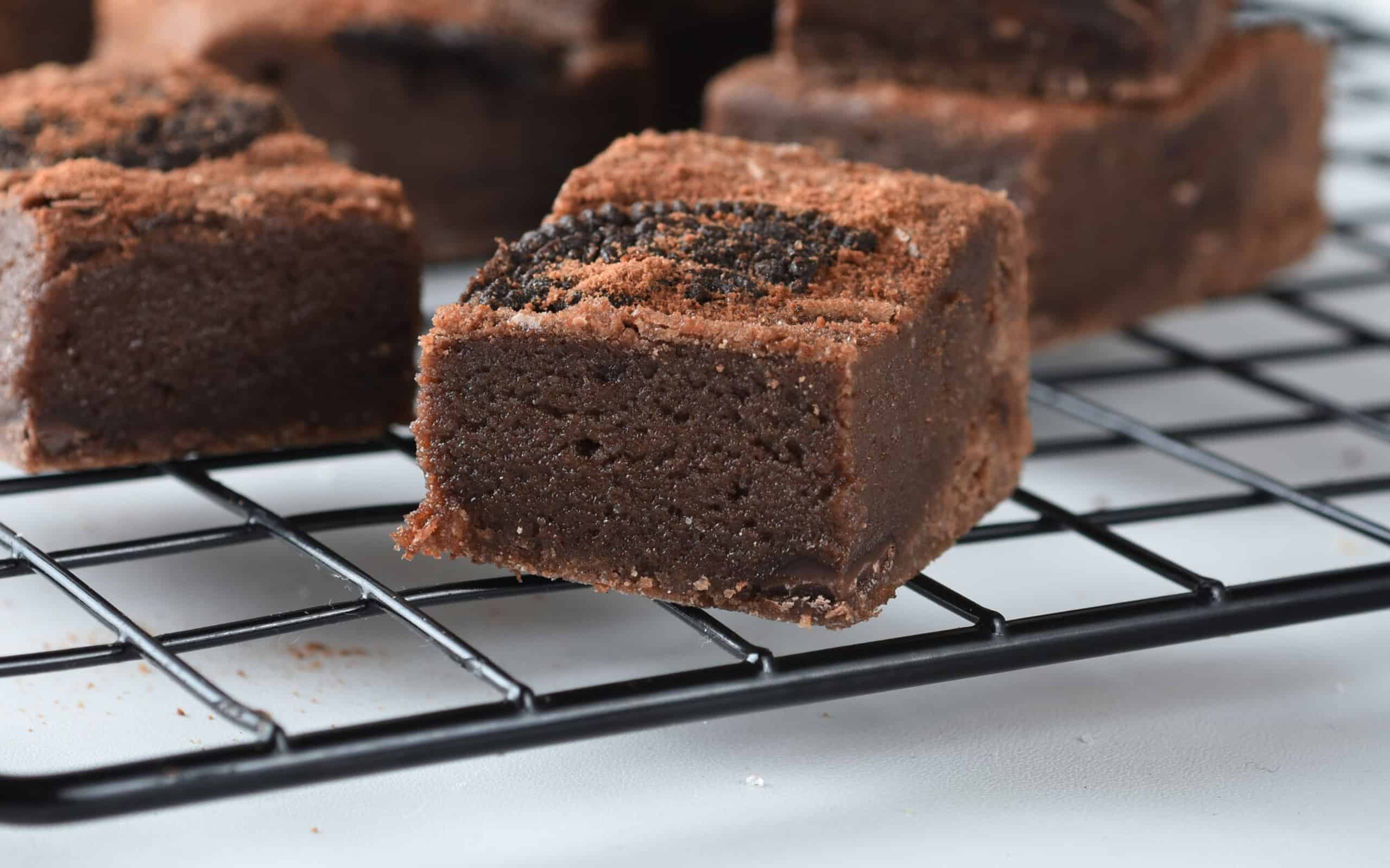
©Edgunn/Shutterstock.com
Ingredients:
* 4 squares unsweetened chocolate
* 3/4 cup margarine
* 2 cups sugar
* 3 eggs
* 1 teaspoon vanilla
* 1 cup flour
* 1 cup nuts, chopped (optional)
* 2 2/3 cups halved strawberries
* 1 tub (8 ounce size) chocolate nondairy whipped topping
Directions:
Heat oven to 350 degrees F (325 degrees F for glass dish). Line a 13 inch by 9 inch baking pan with foil, extending over edges to form handles. Grease foil.
Cook chocolate and margarine in large microwave-safe bowl on high (100 percent power) for 2 minutes or until butter is melted. Stir until chocolate is completely melted.
Stir sugar into chocolate until well blended. Mix in eggs and vanilla. Stir in flour and nuts until well blended.
Spread in prepared pan.
Bake in a 350 degrees F oven for 30 to 35 minutes or until toothpick inserted in center comes out with fudgy crumbs. Do not over-bake. Cool in pan.
Refrigerate 30 minutes for easier cutting. Lift brownies out of pan with foil handles. Invert onto back of pan.
Carefully remove foil. Invert onto cutting board.
Cut into heart shapes with cookie cutter.
Place half of the brownies on individual dessert plates.
Spoon 1/2 cup strawberries over each brownie. Top each with 1/3 cup whipped topping.
Cover with remaining brownies. Garnish with additional strawberries.
Vanilla Cherry Fudge
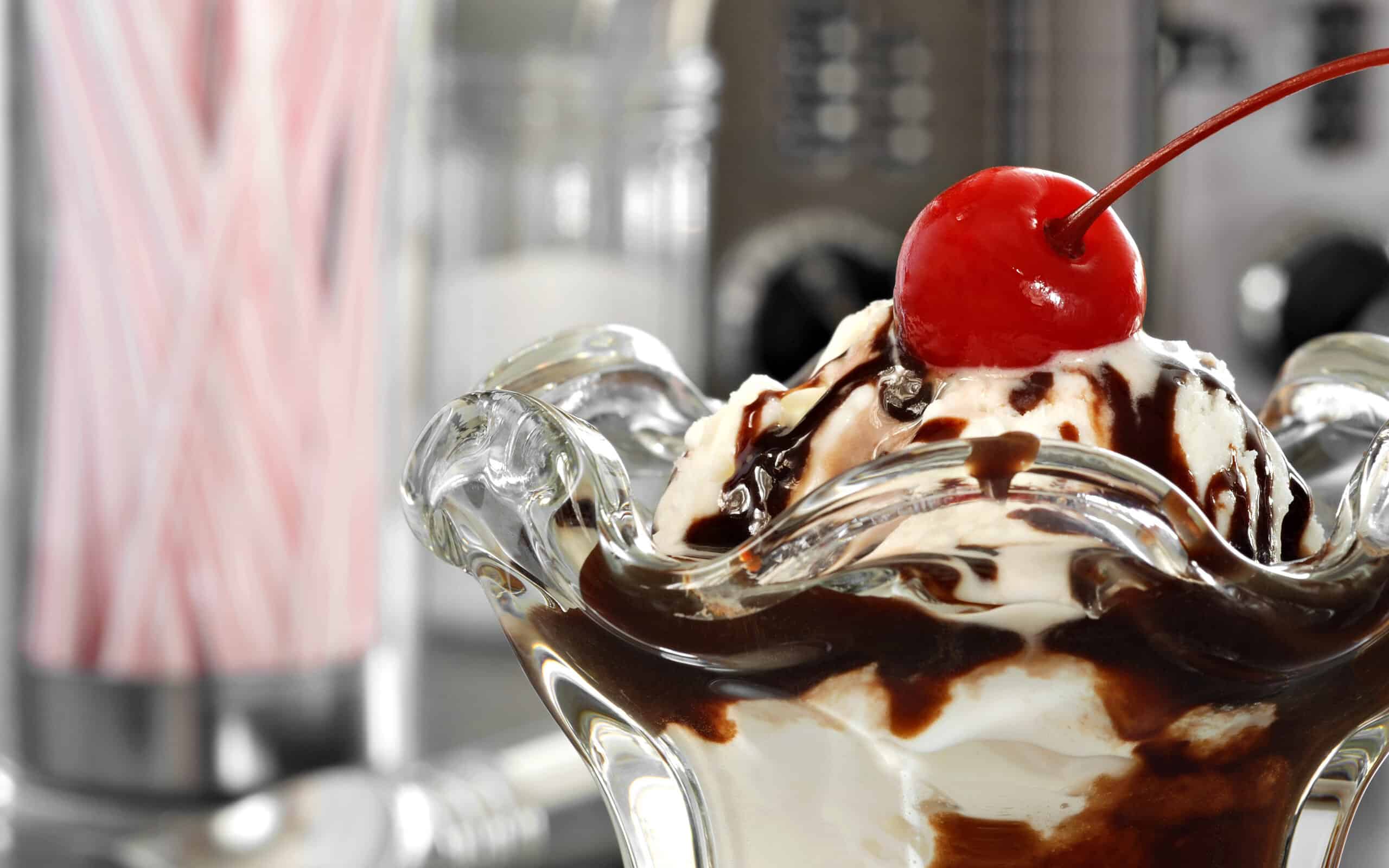
©Marie C Fields/Shutterstock.com
Ingredients:
* 1/2 cup granulated sugar
* 1/2 cup dairy sour cream
* 1/3 cup light corn syrup
* 2 tablespoons butter
* 1/4 teaspoon salt
* 2 teaspoons vanilla
* 1/2 cup quartered candied cherries
* 1 cup coarsely chopped walnuts
Directions:
In 2-quart glass measuring cup, combine sugar, sour cream, corn syrup, butter and salt.
Microwave at high for 5 minutes. Stir until sugar dissolves.
Microwave at High 6 minutes or just until mixture reaches 236 degrees F. (Soft ball).
Let stand 15 minutes without stirring.
Add vanilla, beat until it loses its gloss (about 6 minutes).
Stir in cherries and walnuts.
Quickly pour in buttered 8-1/2 by 4-1/2″ loaf pan. Cool. Cut into squares.
The image featured at the top of this post is ©New Africa/Shutterstock.com.